Microfluidic 3D Printing for High-Resolution Multimaterial Fabrication
This case study highlights a microfluidic 3D printing approach that integrates microfluidics and laser lithography to fabricate high-resolution, heterogeneous 3D structures. By combining multimaterial handling with precise polymerization (down to 10 μm), “3D-FlowPrint” enables the creation of complex micro-devices and culture models.
The system’s material delivery, controlled by Fluigent's Flow-EZ, prevents cross-contamination and facilitates the production of sub-millimeter to millimeter scale objects. Validated with PEGDA-based hydrogels, this microfluidic 3D printer demonstrates significant potential for developing engineered microenvironments for cell culture and advancing bioprinting applications.
Paper from Fluigent and the LAAS – CNRS Group
Paper: Fournié, V.; Venzac, B.; Trevisiol, E.; Foncy, J.; Roul, J.; Assie-Souleille, S.; Escudero, M.; Joseph, P.; Reitz, A.; Malaquin, L. A Microfluidics-Assisted Photopolymerization Method for High-Resolution Multimaterial 3D Printing. Additive Manufacturing 2023, 72, 103629.
This study is the result of a collaboration between the Toulouse Biotechnology Institute, the RESTORE Research Center from the University of Toulouse, the Research laboratory specialized in system analysis and architecture (LAAS) from CNRS and Fluigent. The LAAS develops methodologies for modeling and controlling complex systems. The lab conducts research in biological, micro/nano, and autonomous technologies, driving innovation in healthcare and sustainability. As one of the first “Carnot Institutes” in 2006, the LAAS fosters strong industry collaborations to advance biological research.
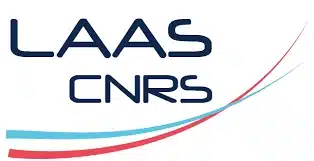
“Establishing our 3D flow-printing platform required fine-tuned flow control and precise synchronization. We wouldn’t have achieved this without the critical support of the Fluigent R&D engineers. Their hands-on involvement helped us improve the reliability and responsiveness of flow modulation. We encountered challenges in stability and reproducibility, but their input led to smart adjustments that made a real difference. From firmware updates to hardware tweaks, their team was reactive and committed. The collaboration extended beyond standard support—it was true co-development. Working closely with Fluigent gave us confidence in our experimental pipeline. We are sincerely thankful for this fruitful and dynamic partnership.”
Dr. Laurent Malaquin, CNRS – LAAS, Research Director
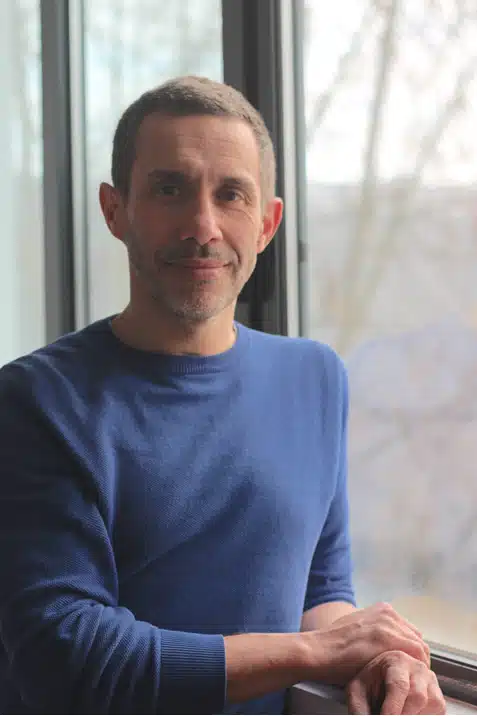
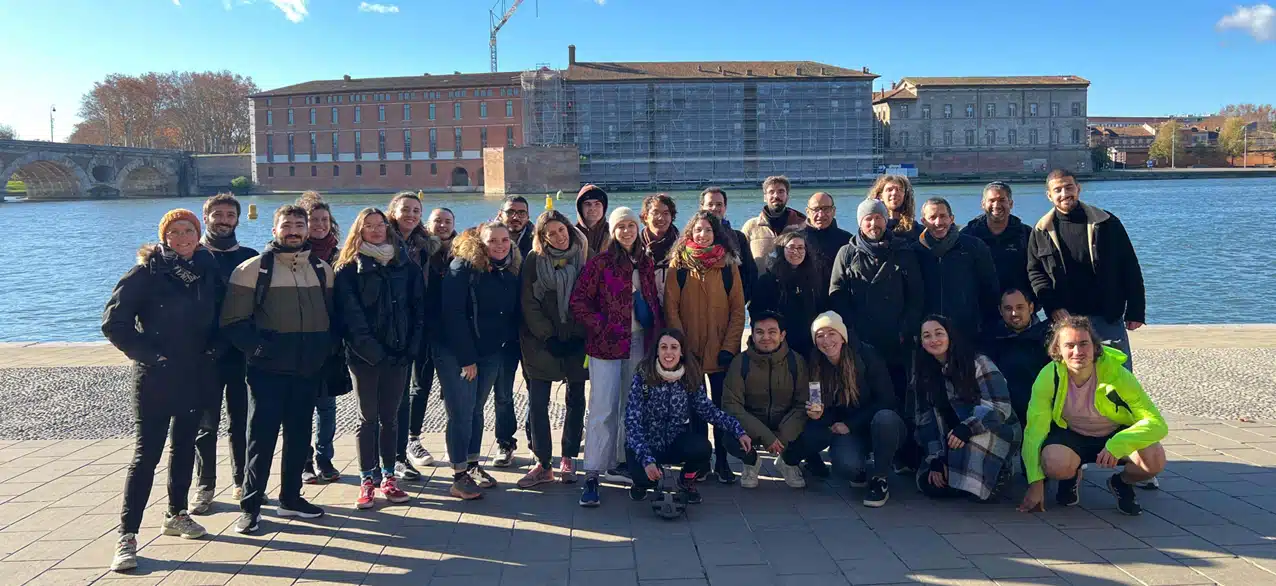
Advantages and Limitations of 3D Printing
3D printing has emerged as a promising technology for fabricating complex biological structures, enabling the precise deposition of biocompatible materials, cells, and hydrogels to create functional tissue models.1,2 Achieving high spatial resolution, on the order of tens of micrometers, while integrating multiple materials within a single construct is essential for developing physiologically relevant models.3,4 Conventional techniques such as micro-extrusion and inkjet printing facilitate multimaterial deposition but are inherently limited in resolution due to nozzle size and droplet formation constraints (Figure 1).5–7 Laser-assisted methods offer higher resolution but are typically restricted to single-material environments, limiting their applicability for heterogeneous structures.8,9 Photolithographic approaches, capable of sub-micrometer resolution, have garnered significant interest due to their ability to decouple material transport from structuring, allowing for highly controlled fabrication.10 However, traditional stereolithography (SLA) systems are predominantly designed for non-biological applications and offer limited multimaterial capabilities.11
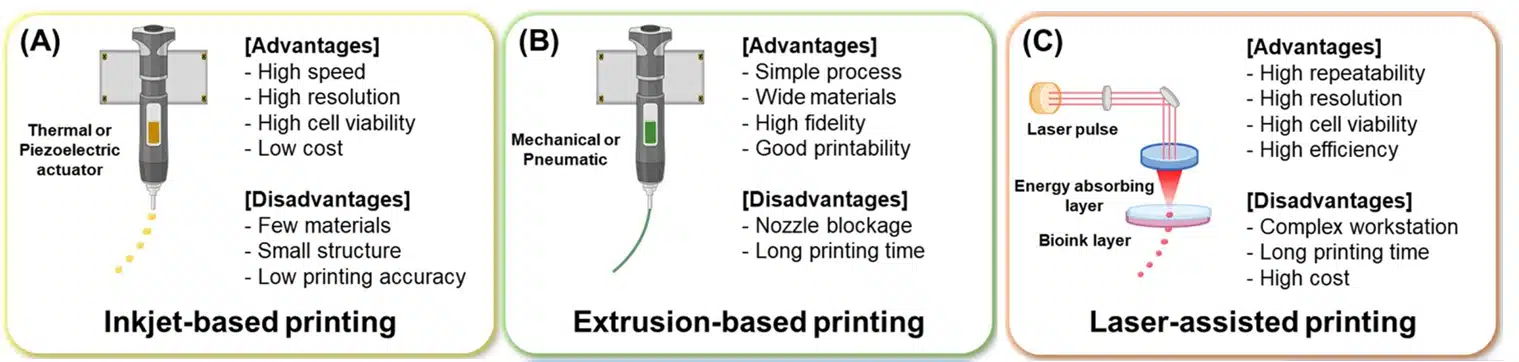
Figure 1: Advantages and limits of classically used 3D bioprinting technologies. (From Kim, J. J. et al.; Virtual and Physical Prototyping, 19(1)).6
How Microfluidics Improves 3D Printing
While 3D printing has advanced microfluidic chip prototyping, microfluidics can enhance 3D printing by enabling precise material delivery, fluid handling, and multimaterial integration. The ability to control material flow at the micro-scale allows for the simultaneous use of multiple materials with varying properties, offering increased flexibility in creating complex structures.12 This flexibility in material selection is particularly valuable for designing intricate 3D objects with diverse characteristics, such as varying stiffness, biocompatibility, and mechanical strength, within a single printed structure (Table 1).13 Despite challenges such as high flow rates, geometric constraints, material recovery, and contamination, microfluidic systems can improve the precision, efficiency, and versatility of 3D bioprinting, offering potential for advanced applications in complex biological structures.14,15
Table 1: Comparison of properties of bioinks for 3D bioprinting technique. (Adapted from Park, W. et al; IJMS 2021, 22 (15), 7837).13
Source | Bioink Type | Mechanical Property | Cytocompatibility | Printability |
---|---|---|---|---|
Natural Polymer | Collagen | Weak, <1 kPa elastic moduli | Cell favorable | Poor printability |
Gelatin | Weak and unstable | Cell favorable | 340-450 µm | |
Fibrin | Weak, ̴50 Pa | Cell favorable | Poor printability | |
Silk Fibroin | Strong, ̴ 25 kPa tensile strength | Non-cytotoxic, Weak cell adhesive | 280-320 µm | |
Alginate | Tunable, varied with molecular weight and Ca2+ contents | Non-cytotoxic, Weak cell adhesive | Poor printability | |
Agarose | Fragile, 3-15 kPA compressive strength | Non-cytotoxic, Weak cell adhesive | >500 µm | |
Synthetic Polymer | Poly(ethylene glycol) | Tunable | Non-cytotoxic, Weak cell adhesive | >200 µm |
Pluronic F127 | Soft and weak | Cytotoxic | ̴ 150 µm |
Aim of the Study
This case study introduces the 3D-FlowPrint concept, a novel printhead design that uses hydrodynamic confinement for efficient microfluidic injections. The system features both aspiration and injection capabilities with adjustable pressures, minimizing contamination risk by recovering excess material. Inspired by the microfluidic probe concept, this microfluidic 3D printing approach allows injection of viscous materials and operation at distances over 500 μm from the substrate.
A key innovation is the integration of an optical fiber for in-situ photopolymerization during material injection, a first for hydrogels. This combination of open microfluidics and integrated optics enables precise control over material composition and multiscale 3D object fabrication.
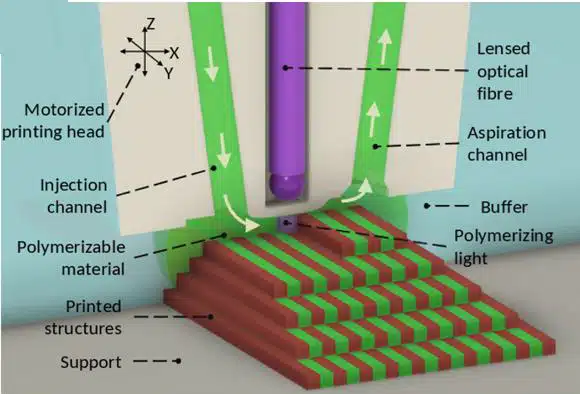
Figure 2: Illustration of the microfluidic 3D printer “3D-FlowPrint”
Methodology: Use of Microfluidics for 3D Printing
The 3D-printed microfluidic printhead integrates a conical design with precision-engineered channels for material injection, aspiration, and optical fiber placement. Fabricated using SLA 3D printing with high-resolution DL260 composite photosensitive resin, the printhead achieves precise microfluidic control and optical integration. The assembled system includes borosilicate glass sealing, PDMS coating for non-adhesive properties, and a ball-lensed optical fiber optimized for photopolymerization. Laser control is achieved using a 405-nm monomodal laser with adjustable power modulation, ensuring precise polymerization. A function generator fine-tunes beam intensity, while fiber positioning adjusts resolution, enabling high-precision or broader polymerization control.
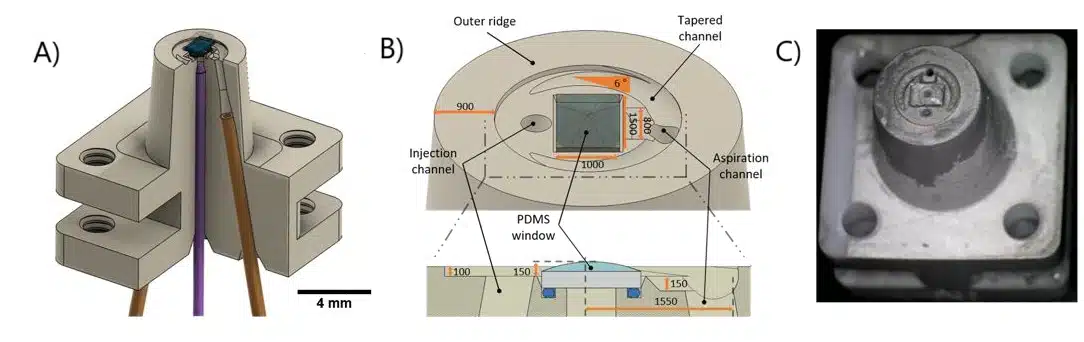
Fluigent’s microfluidic components ensured precise fluid control in this microfluidic 3D printing technique. A M-Switch valve selected solutions, while Flow-EZ and Push-Pull controllers regulated injection and aspiration pressures. Flowrate sensors enabled real-time feedback, maintaining hydrodynamic confinement and preventing cross-contamination by dynamically adjusting flowrates based on printing speed, viscosity, and Zgap. The entire setup is automated through a custom LabVIEW interface, synchronizing fluidic, optical, and mechanical components for seamless operation.
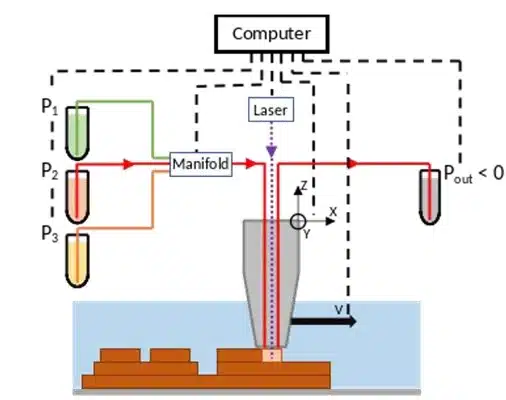
Figure 4: Schematic representation of the microfluidic 3D printer. (From Fournié, V. et al.; Additive Manufacturing 2023, 72, 103629).1
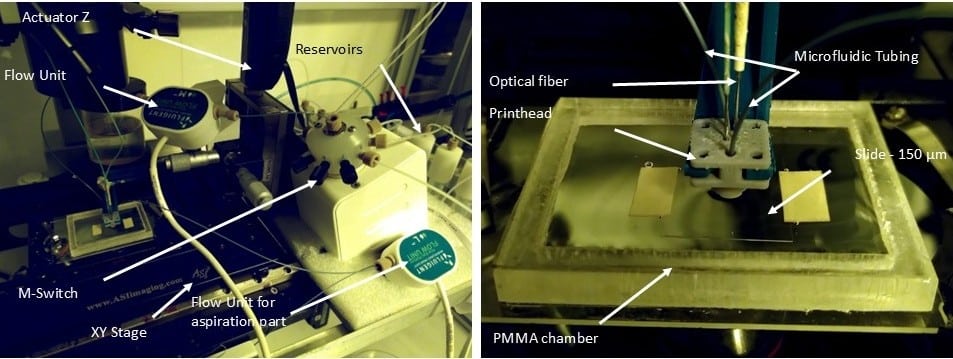
Figure 5: Image of the microfluidic 3D printer.
To validate biological applications, PC3-GFP prostate cancer cells and hASC spheroids were cultured and integrated into polyethylene glycol diacrylate (PEGDA) structures. Cell viability, adhesion, and spatial organization were assessed using fluorescence staining and microscopy, confirming the system’s potential for bio-fabrication and tissue engineering applications.
Proof-of-Concept: High-Precision 3D Printing with Microfluidic-Assisted Photopolymerization
Microfluidic 3D printing combined flow control and photopolymerization to generate highly precise 3D structures. A printhead, similar to microfluidic probes, injected and confined materials onto a substrate, preventing cross-contamination and allowing for multiple materials to be printed (Figure 5). PEGDA hydrogels were successfully used, solidified by a light-sensitive chemical (LAP photo-initiator) and a focused 405 nm laser, enabling micron-level precision that classical methods couldn’t match.
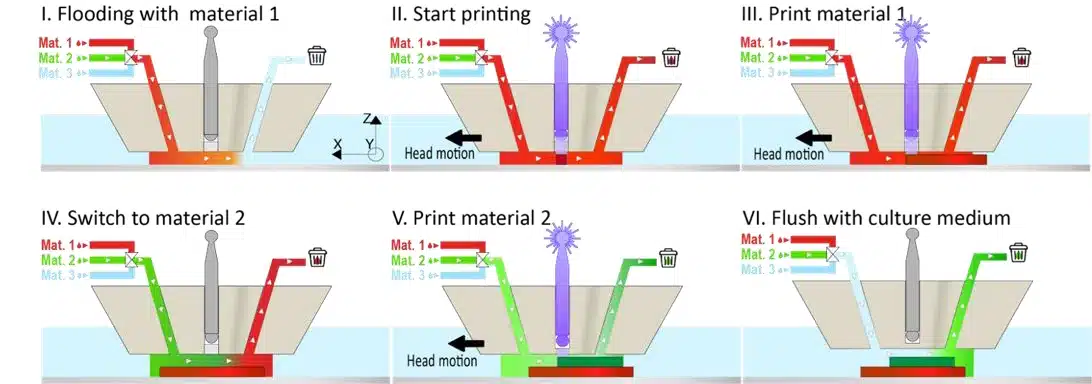
Figure 6: Schematic representation of the multimaterial printing process.1
Hydrodynamic Flow Confinement
Controlling material flow is key to preventing contamination, especially with multimaterial printing. The printhead featured a small gap between it and the substrate, creating hydrodynamic resistance to direct material toward the aspiration channel. A tapered channel and peripheral ridge ensured the injected material stayed contained. CFD simulations and experiments confirmed that this design effectively controlled the material flow, achieving high-resolution prints without contamination (Figure 6).
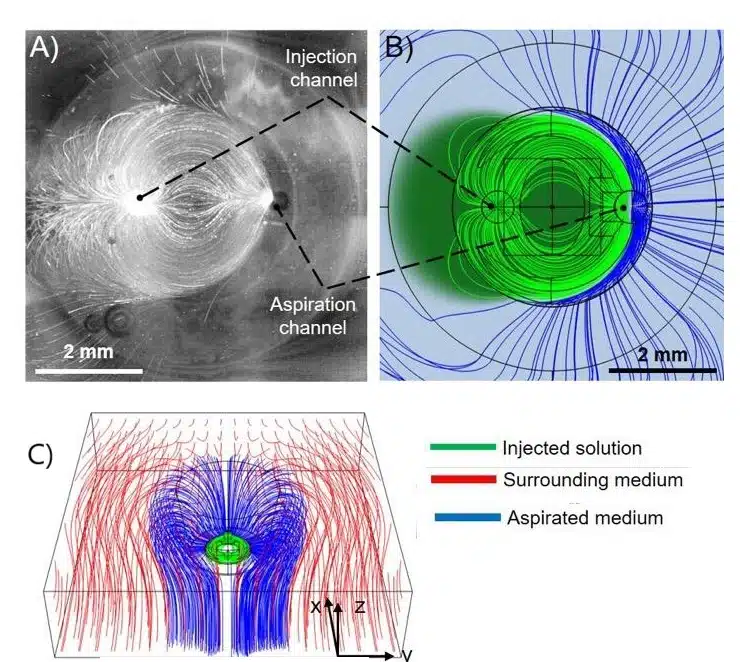
Figure 7: A) Experimental analysis showing the simultaneous injection and aspiration of a suspension of fluorescent particles. (B) Comsol simulations performed according to experimental parameters. C)3D flow distribution obtained from Comsol simulations.1
Layer Thickness Control
Layer thickness control in the 3D-FlowPrint is controlled by the Zgap, defined as the confinement between the PDMS window and the substrate. Experimental validation showed that structures printed at different Zgap values (60–350 μm) maintained consistent lateral dimensions (~30 μm), indicating minimal light spread (Figure 7). Confocal imaging revealed a 16% shrinkage along the Z-axis, with slight slopes at line edges. The low absorbance of PEGDA-based hydrogels (≤0.01 m⁻¹) ensured uniform polymerization, allowing for precise layer formation.
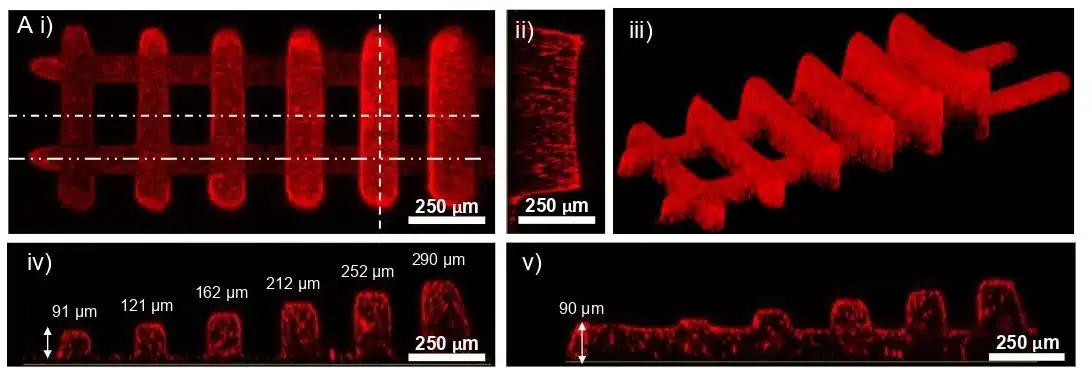
Figure 8: Confocal pictures of a ladder structure with segments printed at varying heights.1
Multimaterial Printing Capabilities
Microfluidic 3D printing allows real-time switching between materials, creating complex structures without cross-contamination. For example, PEGDA solutions with fluorescent nanoparticles produced clear, sharp features. Controlled flushing and gentle flow ensured material separation, enabling the fabrication of complex multimaterial structures with minimal stress (Figure 8).
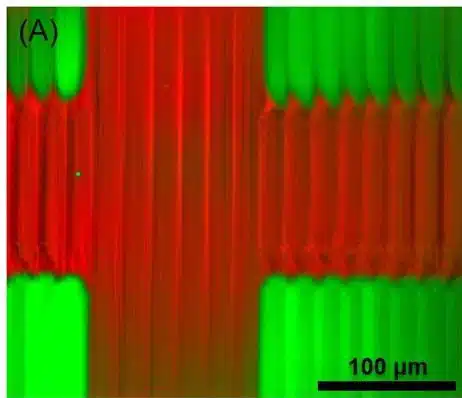
Cell Patterning and Spheroid Engineering
The experiments done with the microfluidic 3D printer demonstrated precise cell patterning with non-adherent PEGDA structures. PC3-GFP cells were seeded onto printed arrays, including lines, grids, and converging lines. No cell adhesion occurred on the PEGDA patterns. By day 3, cell proliferation resulted in confluence (Figure 9). This technique also allowed for spatial control of human ASC spheroid migration using PEGDA patterns with guiding channels of varying widths (100–200 μm). After 3 days, spheroid sprouting was restricted to the adhesive regions, demonstrating the system’s ability to control cell behavior. These results suggest the potential of microfluidic 3D printing for studying confined migration, cancer invasion, and for applications in tissue engineering.
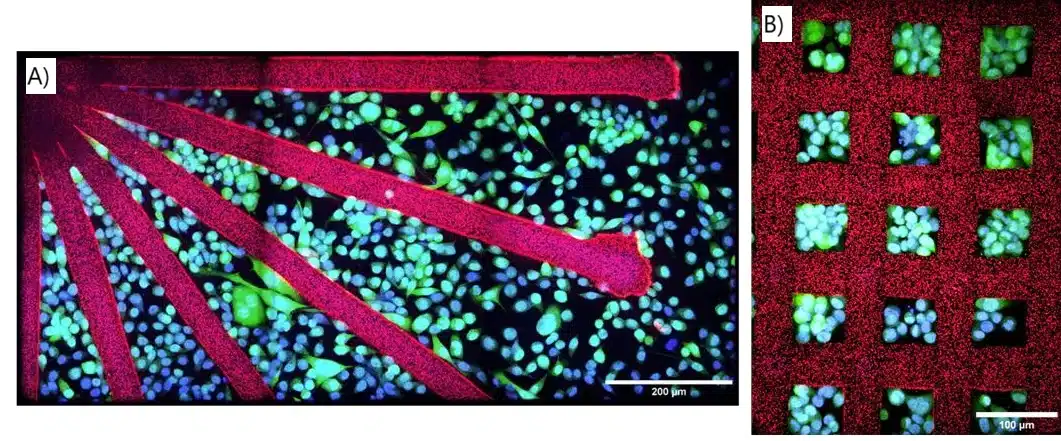
Conclusion
In this study, researchers from Fluigent and the LAAS-CNRS developed a microfluidic 3D printing method that combines photopolymerization with microfluidic flow control to create high-resolution, multimaterial structures. By preventing cross-contamination, this approach enables precise material delivery directly onto the substrate. The optimized printhead design, validated through simulations and experiments, ensures effective material confinement, allowing for precise, high-resolution fabrication. The system’s versatility was demonstrated through the successful creation of complex structures, including those for cell culture applications. Future advancements should focus on expanding the range of printable biomaterials, particularly for 3D bioprinting, to support tissue engineering and other biomedical applications.
Related Products
Expertises and resources
-
Microfluidics Case Studies Microfluidic Transistor for Precise Fluid Control Read more
-
Microfluidics White Papers An exploration of Microfluidic technology and fluid handling Read more
-
Expert Reviews: Basics of Microfluidics The Importance of Flow Control Stability in Microfluidics Read more
-
Expert Reviews: Basics of Microfluidics Flow Control Technologies: Comparison between peristaltic, syringe and pressure pumps for microfluidic applications Read more
-
Expert Reviews: Basics of Microfluidics Microfluidic Resistance Read more
References
1. Fournié, V. et al. A microfluidics-assisted photopolymerization method for high-resolution multimaterial 3D printing. Addit. Manuf. 72, 103629 (2023).
2. Daly, A. C., Prendergast, M. E., Hughes, A. J. & Burdick, J. A. Bioprinting for the Biologist. Cell 184, 18–32 (2021).
3. Ozbolat, I. T. & Hospodiuk, M. Current advances and future perspectives in extrusion-based bioprinting. Biomaterials 76, 321–343 (2016).
4. Ravanbakhsh, H. et al. Emerging Technologies in Multi‐Material Bioprinting. Adv. Mater. 33, 2104730 (2021).
5. Ning, L. & Chen, X. A brief review of extrusion‐based tissue scaffold bio‐printing. Biotechnol. J. 12, 1600671 (2017).
6. Kim, J. J. & Cho, D.-W. Advanced strategies in 3D bioprinting for vascular tissue engineering and disease modelling using smart bioinks. Virtual Phys. Prototyp. 19, e2395470 (2024).
7. Gudapati, H., Dey, M. & Ozbolat, I. A comprehensive review on droplet-based bioprinting: Past, present and future. Biomaterials 102, 20–42 (2016).
8. Devillard, R. et al. Cell Patterning by Laser-Assisted Bioprinting. in Methods in Cell Biology vol. 119 159–174 (Elsevier, 2014).
9. Hakobyan, D. et al. Laser-Assisted Bioprinting for Bone Repair. in 3D Bioprinting (ed. Crook, J. M.) vol. 2140 135–144 (Springer US, New York, NY, 2020).
10. Zheng, Z. et al. Visible Light-Induced 3D Bioprinting Technologies and Corresponding Bioink Materials for Tissue Engineering: A Review. Engineering 7, 966–978 (2021).
11. Ge, Q. et al. Projection micro stereolithography based 3D printing and its applications. Int. J. Extreme Manuf. 2, 022004 (2020).
12. Miri, A. K. et al. Microfluidics‐Enabled Multimaterial Maskless Stereolithographic Bioprinting. Adv. Mater. 30, 1800242 (2018).
13. Park, W., Gao, G. & Cho, D.-W. Tissue-Specific Decellularized Extracellular Matrix Bioinks for Musculoskeletal Tissue Regeneration and Modeling Using 3D Bioprinting Technology. Int. J. Mol. Sci. 22, 7837 (2021).
14. Han, D., Yang, C., Fang, N. X. & Lee, H. Rapid multi-material 3D printing with projection micro-stereolithography using dynamic fluidic control. Addit. Manuf. 27, 606–615 (2019).
15. Lipkowitz, G. et al. Injection continuous liquid interface production of 3D objects. Sci. Adv. 8, eabq3917 (2022).