UV-C and microfluidics for biofilm studies as a new experimentation method
This article, published in Biomicrofluidics 2023, is the result of a collaboration between the Institute of Fluid Mechanics and the Laboratory of Chemical Engineering from the University of Toulouse and the CNRS (France).
It presents a microfluidic approach coupled with UltraViolet-C (UV-C) emission as a new method for studying bacterial biofilms, integrating Fluigent products in its experiments. This allows users to obtain homogeneous results thanks to a controlled fluid flow, generated by Fluigent’s MFCS™ series. The results obtained make microfluidics an ideal model for meeting the challenge of understanding biofilms, particularly in the presence of UV-C, which can be used to control biofilm formation in a precise zone of interest, thereby increasing the duration of experiments.
A paper from the Toulouse Institute of Fluid Mechanics
Ramos, G.; Toulouze, C.; Rima, M.; Liot, O.; Duru, P.; Davit, Y. Ultraviolet Control of Bacterial Biofilms in Microfluidic Chips. Biomicrofluidics 2023, 17 (2), 024107. https://doi.org/10.1063/5.0135722.
The institute of Fluid Mechanics (University of Toulouse – CNRS) specializes in three main fields of application: engineering, life mechanics, and environmental fluid mechanics. Since the 1980s, the institute has continuously developed new research topics, including microfluidics, and publishes about a hundred papers per year. One of the institute’s many areas of expertise is porous and biological media, and the study of biofilms helps to characterize these environments. Under the leadership of fluidic expert Dr. Paul Duru, their research is at the interface between health and the environment, and they use microfluidic tools to carry out their projects.
Researchers from IMFT, led by Dr. Yohan Davit and in collaboration with the Laboratory of Chemical Engineering, have developed a new, reliable, and representative approach using UV-C emissions combined with microfluidics for biofilm studies.
This work is part of an ERC Sarting Grant and experiments were performed at the BioPorousLab.
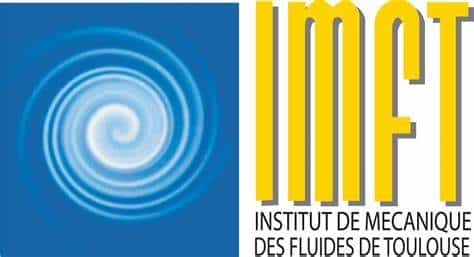
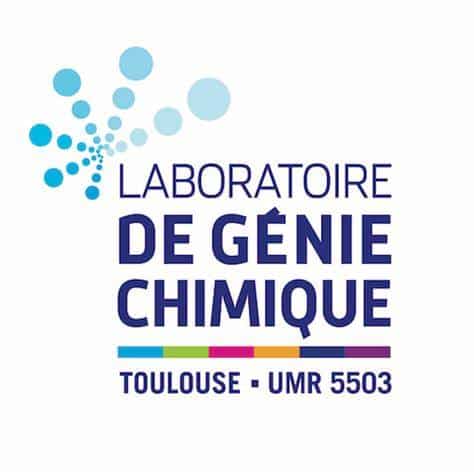
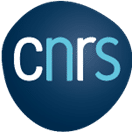
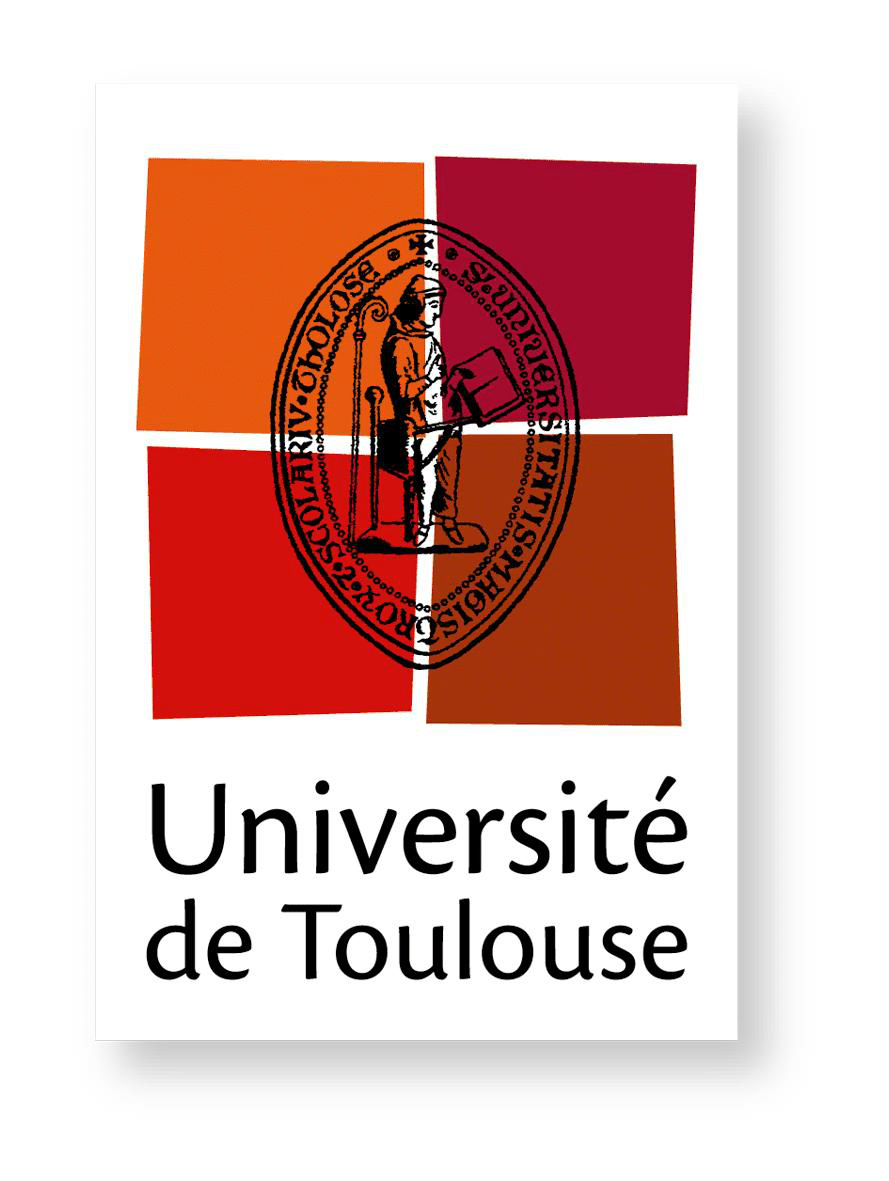

What is a bacterial biofilm?
All microorganisms have conditions that favor their development and growth. When their optimal conditions are no longer respected, or when they feel attacked by antibiotics or the immune system, bacteria leave their planktonic form to create a biofilm adhering to a support and become sessile (Figure 1).
Biofilms also constitute a set of cellular networks in which cells communicate with each other, adapt to their environment by modifying their gene expression. Environmental changes and chemical communication between cells enable the uptake and production of signaling molecules. These mechanisms give rise to an adaptation known as quorum sensing.1 bacteria synthetize molecules called Auto-Inducers (AI). When these molecules reach a certain threshold, they activate an AI regulator which is also a transcription factor, to form a complex that regulates gene expression.2
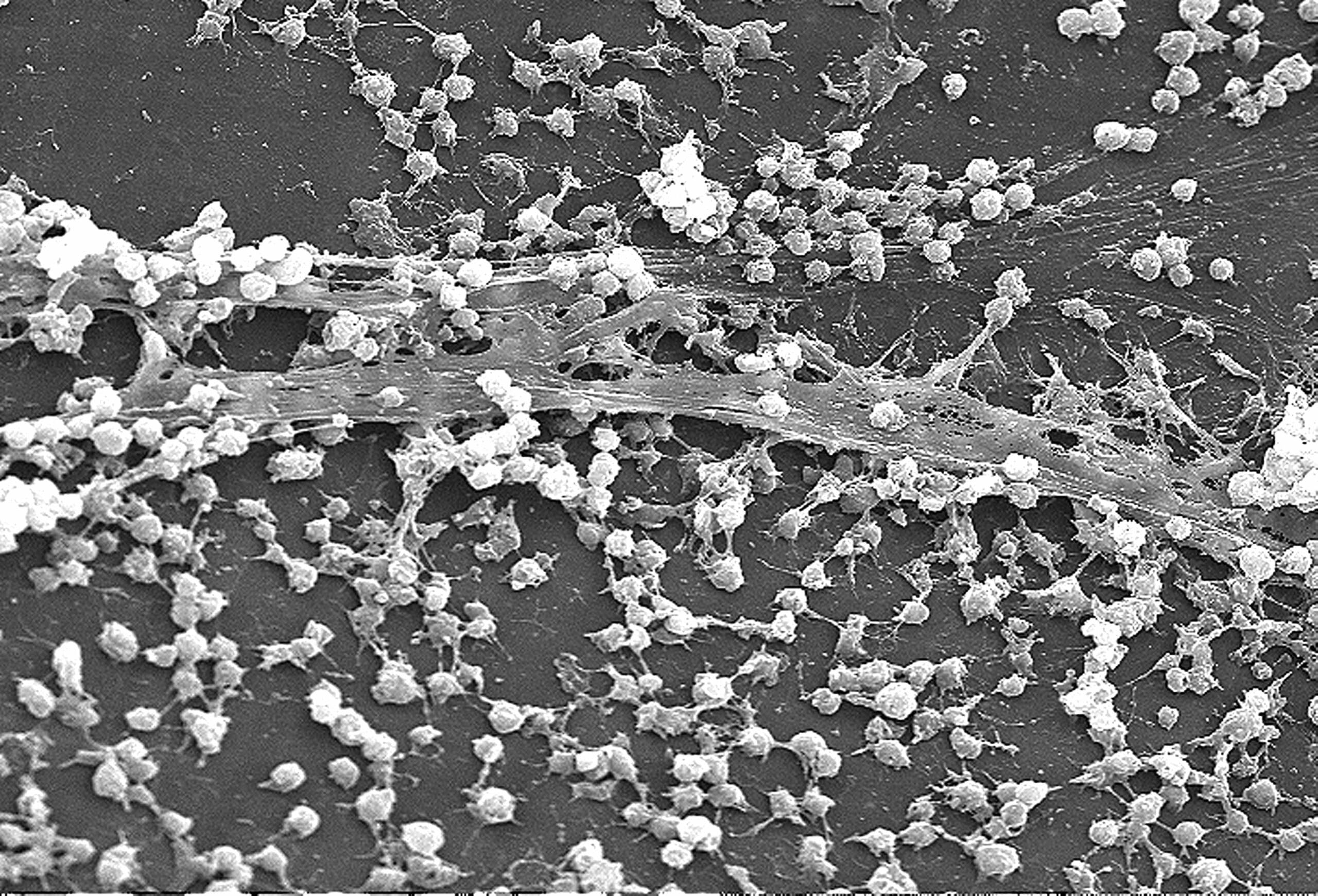
Thanks to quorum sensing, bacterial biofilm can settle on any surface and resists a wide range of treatments. Consequently, they are the source of real industrial and health problems that cause many serious or acute infections. They are also a source of other problems described in the following table (Table 1).3 In addition to affecting numerous societal and industrial factors, biofilms also impact the economic performance of various sectors, costing $5 trillion per year (Figure 2).4
Studying bacterial biofilms is essential for a better understanding of their formation mechanisms to control their progression and limit their negative impacts. However, their complexity makes it difficult to culture and study their resistance mechanisms.
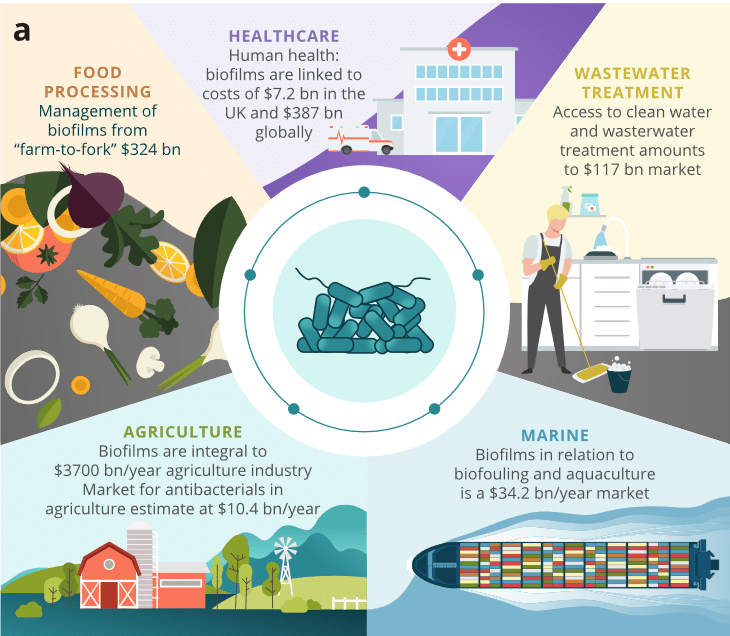
Figure 2. Biofilms impact on different sectors of activity.4
Problem | Antibiotic resistance | Immune system resistance | Industrial problems |
Reasons – Explications | Modification of their physiological properties | – Their size prevents phagocytosis – Extracellular matrix prevents antibodies action | – Pipe colonization – Biofilm formation on ship hulls – Food spoilage |
What we already know about their formation
The formation of this biofilm takes place in several stages, forming a two-phase cycle, reversible or irreversible, each consisting of different distinct stages: motility, adhesion, maturation, dispersal, and propagation of the biofilm.5 During biofilm formation, bacteria secrete polymers called exopolysaccharides or EPS (Extracellular Polymeric Substances) to form a protective extracellular matrix (Figure 3).
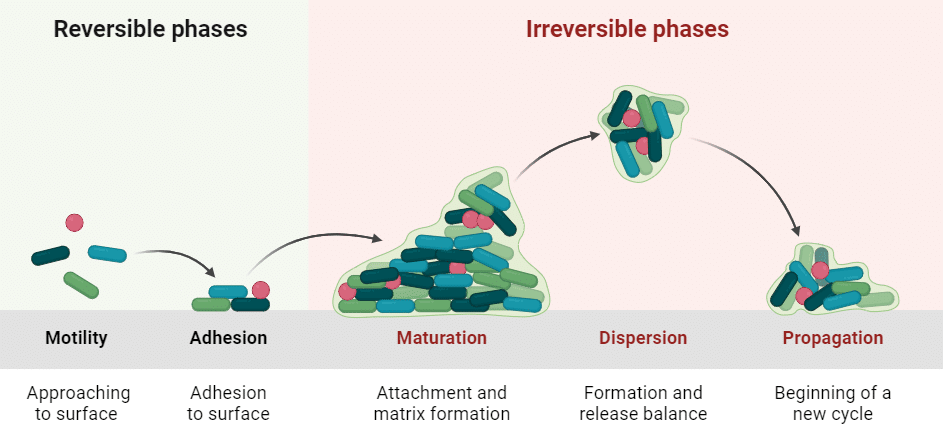
Figure 3. Biofilm formation cycle.6
Current methods of bacterial biofilm studies
To better understand their mechanisms, several experimental approaches have been developed to study biofilms. The traditional method is microtiter plate culture followed by staining. It is based on the principle of incubating bacteria on a plate to promote their growth. Growth is then measured by absorbance after the addition of crystal violet (Figure 4).3,7 Although this technique is fast, it has a few drawbacks. For example, it is difficult to reproduce all the environmental conditions conducive to biofilm development with this study model. This model provides results at a fixed point in time, preventing the analysis of biofilms over a long period of time, complicating our understanding of their mechanisms. With the microtiter plate method, we can’t observe and study early and mature biofilms, which are best associated with microfluidic systems.8,9

Figure 4. Traditional method to culture biofilms by microtiter plates and crystal violet.7
Microfluidic for biofilm studies
Microfluidics appears to be a promising approach for studying bacterial biofilms, offering solutions to problems classically encountered with other techniques. It can reproduce the physiological conditions required for biofilm development, due to its precise flow control. Also, microfluidic chips can imitate chemical, physical, and biological variations as occurred in a natural environment.8 This is particularly true for flow-cell culture (Figure 5).
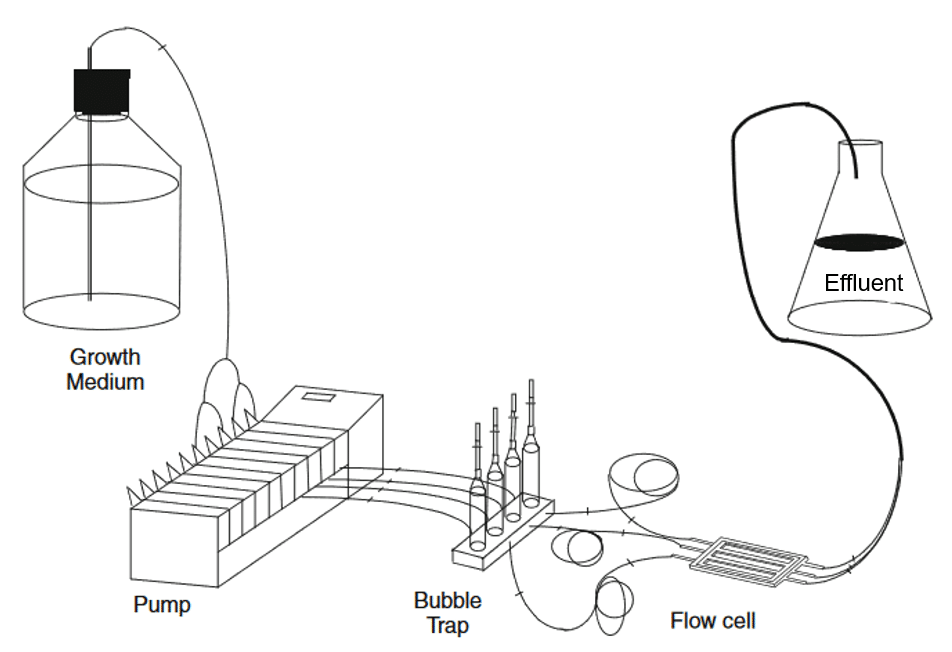
Device | Application | Advantages | Limitations |
Microtiter plate | – Screening for biofilm formation capacity. – Test of anti-biofilm. compounds. | – High-Throughput. Inexpensive. – No need for advanced equipment apart from plate reader. – Dedicated microscopic-grade microplates allow noninvasive imaging. | – Loosely attached biofilm may not be measured correctly (can be detached during washing steps). – Sensitive to sedimentation. – End-point measurement. – Match mode. – Exhaustion of nutrients. – Direct inspection difficult – Usually only short-term experiments. – Possible interference with liquid-air pellicle. – Sometimes poor reproducibility Results person or laboratory dependent. – Assessment possible only at sufficiently high cell density. – Not suitable for investigating early stages of biofilm formation. |
Microfluidics | It can be designed for special purposes, e.g, mimicking air-liquid interfaces, provide in situ mixing of reagents, include customized measuring devices. | – Can be custom made for specific purposes. – Versatile. – Compatible with single cells analysis. | – Requires special equipment for manufacturing and running systems. – It can be expensive. – Operation can be tedious. – Clogging can occur due to small dimensions. |
Aim of the study
To take the study of biofilm via microfluidics a step further, researchers from the Institute of Fluid Mechanics demonstrated the possibility of confining biofilms using UltraViolet-C (UV-C) in a specific location of interest within a microfluidic chip; thus, maintaining initial channel flow for longer experiments.
In this bacterial biofilm study, different configurations are tested to prove the efficacy of UV-C against biofilm development, integrating a Fluigent MFCS™ series and Flow Unit. The aim here was to limit the biofilm formation outside the zone of interest.
Control of biofilm formation in microfluidic chips
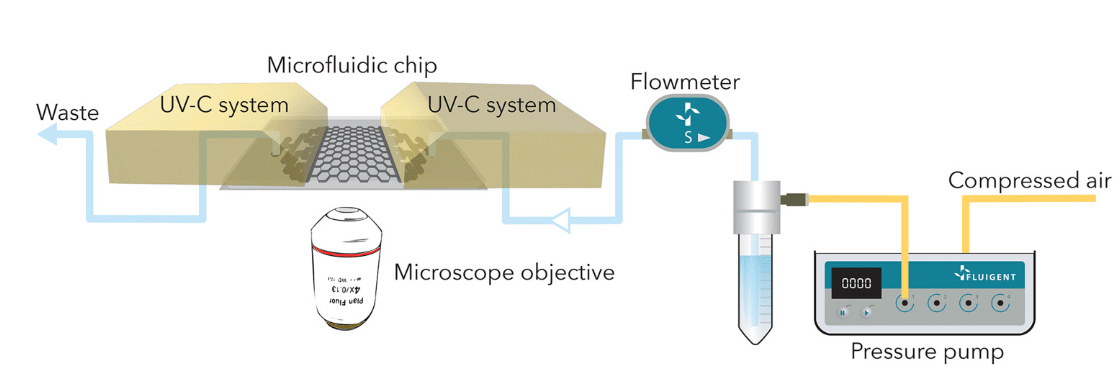
To study and culture bacterial biofilm, a microfluidic chip was made of PDMS, which contained inlet and outlet mixing zones. At the center of the chip, a network of channels was designed in a « honeycomb » pattern, where biofilms were formed. The microfluidic chip was observed using a Nikon Eclipse Ti2-E inverted microscope, x4 objective, and imaged by an sCMOS camera. Microscopic images of the chip were captured automatically every hour.
The MFCS™ series pressure pump generated a constant fluid flow rate of the culture medium, which was controlled by the Fluigent Flow Unit S. Both instruments were connected to Fluigent software (Oxygen, latest version of A-i-O) to adapt the pressure according to the required flow rate (Figure 6). Together, the MFCS series and Flow Unit enabled precise distribution of a culture medium at a flow rate of 2 µL/min to the microfluidic chip, where GFP-coupled Pseudomonas aeruginosa bacteria have been pre-inoculated at a flow rate of 8 µL/min.
The PDMS used for the chip is a material known for its many advantages but is unable to isolate biofilms in an area of interest. Here, to limit biofilm formation at the inlet and outlet of the chip, UV-C was projected onto a mirror to reflect the rays onto a microfluidic chip in the following configuration (Figure 7).
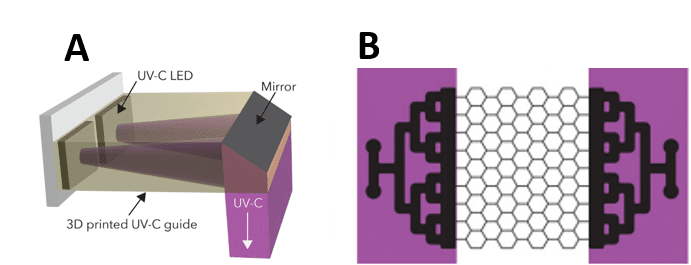
Figure 7. (A) UV-C emission device on mixing areas. (B) Areas of the chip (purple) receiving UV-C emissions
Results: UltraViolet-C system limits biofilm formation in mixing zones.
The biofilm in the microfluidic chip was first observed with and without the presence of UV-C at the mixing zones by measuring the intensity of the GFP and by visualization in bright field (gray levels) microscopy. The presented results were obtained after 48 hours of cultivation within the microfluidic chip.
The B configuration, which was subjected to UV-C at the mixing zones, has significantly less biofilm at the input and output of the chip, unlike the A configuration, where biofilms were developed on the entire chip (Figure 8.A). In the B configuration, especially on the bright field images, we can see that biofilm has formed at the output (Figure 8.B).
This is due to the movement of the biofilm from the area of interest towards the outlet, which are more resistant to UV-C than the bacterium in its planktonic form. The number of biofilms created remained minimal compared to the A configuration without lightning, proving the effectiveness of UV-C.
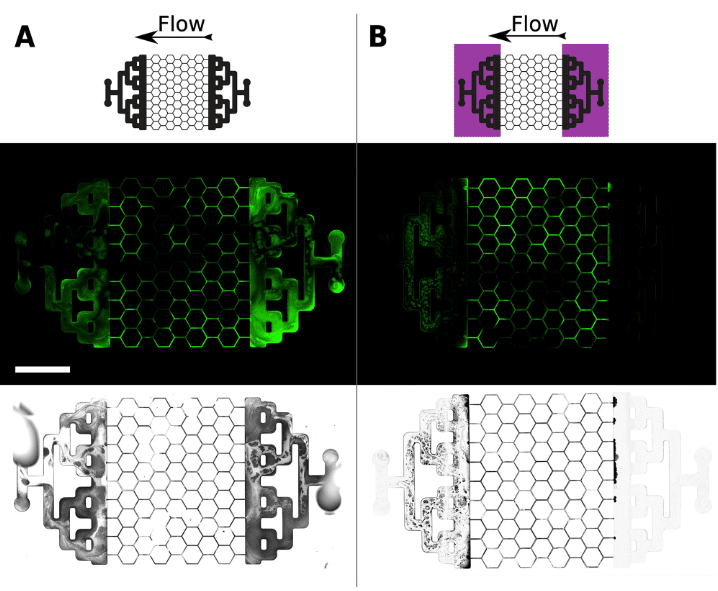
Figure 8. Biofilm formation after 48 hours of culture in the presence (B) or not (A) of UV-C
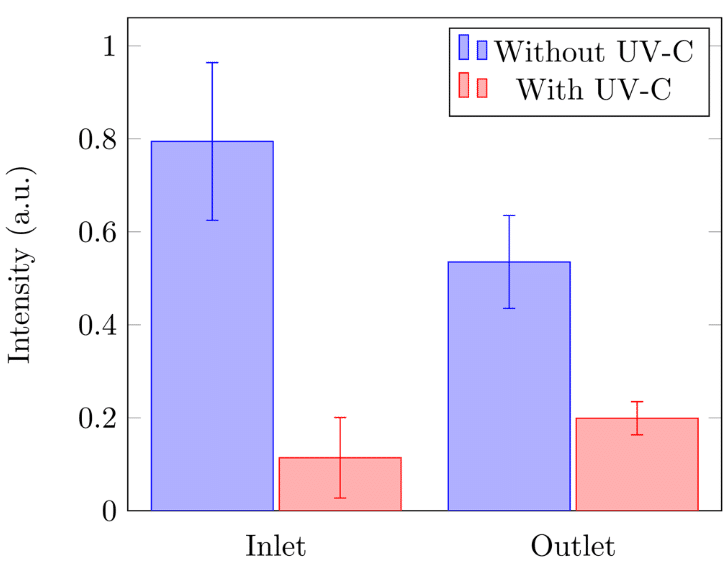
The relative intensity of the GFP (in arbitrary units) confirms the microscopic images obtained. This intensity was measured at the inlet and outlet of the microfluidic chip with and without the presence of UV-C (Figure 9). Whether at the inlet or outlet, the biofilm formation was significantly higher without exposure to UV-C. These results once again showed that after exposure to UV-C, the presence of biofilm in the microfluidic chip was more important at the outlet.
Regarding the flow, the passage of fluids was followed by tracking the particle’s velocimetry (Figure 10). The results showed that without UV-C, the culture medium flowed only within a single channel of the microfluidic chip, as well as in the two mixing zones. The formation of biofilm at these two zones prevents the uniform diffusion of the flow within the area of interest, forming a single distribution channel. Meanwhile, in the case of exposure to UV-C, the culture medium was able to flow within several channels and at the mixing zones, thus proving the effectiveness of UV-C as blockers of biofilm formation.
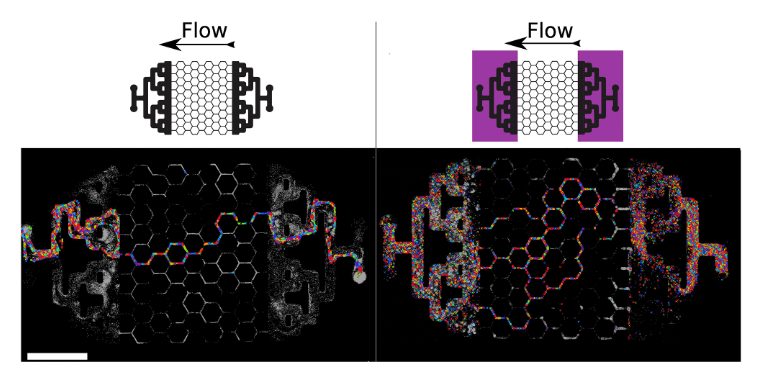
Finally, in addition to allowing a better flow distribution within the chip, the effectiveness of UV-C in long-term experiments was evaluated (Figure 11). The same experiment as in Figure 8 was reproduced but for longer culture times (5 and 7 days). The results obtained were like Figure 8. In the presence of UV-C, biofilm formation at the mixing zones was limited. Even though some biofilms are present at the outlet, due to their displacements from the area of interest to the outlet, no biofilms were found at the inlet even after a week.
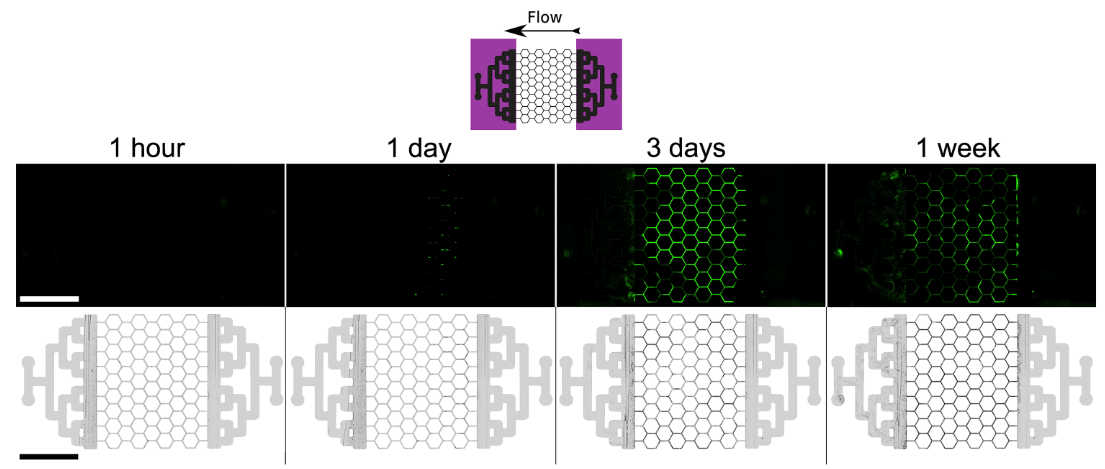
Testimonial
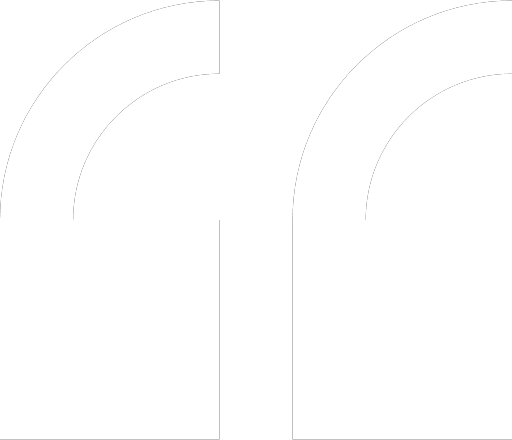
“Our lab uses microfluidics to study bacteria in a range of different conditions. We are trying to understand how biofilm-forming organisms, such as Pseudomonas aeruginosa or Staphylococcus aureus, interact with flows; what type of spatio-temporal dynamics results from couplings between growth, transport mechanisms and flow-induced detachment; and how ecological interactions, such as predation or competition for nutrients, affect these dynamics.
Microfluidic experiments involving bacteria are unforgiving: the smallest flaw in the equipment, design, protocols or realization will likely result in a complete failure. Biological organisms further demand strong statistics, with systematic replication of long and difficult experiments.
We use the pressure pumps and flow sensors from Fluigent to ensure precise and reliable control of the flow conditions over a broad range of flow rates and pressures. The feedback control between the pressure pump and the flow sensor makes it possible to easily switch between imposing a pressure difference, while measuring the flow rate, or imposing the flow rate, while measuring pressure fluctuations. The stability of Fluigent equipment, combined with our UVC system or automatized microscopy, has also made it possible to measure the dynamics of biofilm development over week-long experiments and paves the way towards even longer experiments.”
Yohan Davit, PhD – IMFT
Conclusion
In this paper, researchers from The Toulouse Institute of Fluid Mechanics (University of Toulouse – CNRS) highlighted the study of biofilm via microfluidics as a new model for experimentation. The use of Fluigent products, including the MFCS™ series pump and Flow Unit, has contributed to reproducible and consistent results. Selecting suitable materials for microfluidics is essential for carrying out a quality experiment. They have implemented a technique that frames the development of these biofilms within the area of interest, allowing not only a controlled flow of fluids within the chip, but also allowing the increase of experimental times to mimic biofilm mechanisms. This microfluidic method seems to be the most suitable for the study of biofilms, especially when coupled with UV-C as in this case. New experimentations could pave the way for new applications of microfluidic for biofilm studies.
Related products
Expertises & Resources
References
1. Mukherjee, S. & Bassler, B. L. Bacterial quorum sensing in complex and dynamically changing environments. Nat. Rev. Microbiol. 17, 371–382 (2019).
2. Sharma, A., Singh, P., Sarmah, B. K. & Nandi, S. P. Quorum sensing: its role in microbial social networking. Res. Microbiol. 171, 159–164 (2020).
3. Roux, A. & Ghigo, J.-M. Les biofilms bactériens. Bull. Académie Vét. Fr. 159, 261–268 (2006).
4. Highmore, C. J. et al. Translational challenges and opportunities in biofilm science: a BRIEF for the future. Npj Biofilms Microbiomes 8, 68 (2022).
5. Rather, M. A., Gupta, K. & Mandal, M. Microbial biofilm: formation, architecture, antibiotic resistance, and control strategies. Braz. J. Microbiol. 52, 1701–1718 (2021).
6. Biofilm Formation Cycle by BioRender adapted to Crouzet, M. et al. Exploring early steps in biofilm formation: set-up of an experimental system for molecular studies. BMC Microbiol 14, 253 (2014).
7. Campo-Pérez, V., Alcàcer-Almansa, J., Julián, E. & Torrents, E. A High-Throughput Microtiter Plate Screening Assay to Quantify and Differentiate Species in Dual-Species Biofilms. Microorganisms 11, 2244 (2023).
8. O’Toole, G. A. Microtiter Dish Biofilm Formation Assay. J. Vis. Exp. JoVE 2437 (2011) doi:10.3791/2437.
9. Azeredo, J. et al. Critical review on biofilm methods. Crit. Rev. Microbiol. 43, 313–351 (2017).