Microfluidic Artificial Skin Platform for Sweat Study and Product Development
This case study, based on a publication from Moonen, E.J.M. et al. (Lab On a Chip, 23, 2268–2275), presents a new model to mimic skin via microfluidics, that enables to test new wearable sweat sensing patches during their development. It imitates several characteristics of skin for sudation research like skin typography, wetting properties, and the number of active sweat pores. The artificial skin platform can cover all sweat ranges to mimic the perspiration of both individuals at rest and strenuous exercisers. The Fluigent flow controller and flowsensor permit a precise distribution of sweat in artificial skin.
A Paper From the Department of Mechanical Engineering of the Netherlands
Moonen, E.J.M., Ul Islam, T., Van Kemenade, S., Pelssers, E., Heikenfeld, J., Den Toonder, J.M.J., 2023. A versatile artificial skin platform for sweat sensor development. Lab Chip 23, 2268–2275.
This article, published in Lab On a Chip 2024, is the result of a collaboration between the Department of Mechanical Engineering and the Institute for Complex Molecular Systems (ICMS) at Eindhoven University of Technology, the Philips Research (Royal Philips, High Tech Campus, AE Eindhoven) in the Netherlands, and the Novel Devices Laboratory (Biomedical Engineering Dept., Univ. of Cincinnati, Ohio, USA).
The Eindhoven University of Technology is divided into nine departments. The Department of Mechanical Engineering (ME) is one of them. It aims to conduct world-class research on key topics relevant to high-tech industries in the Netherlands, particularly in Brainport Eindhoven. ME provides an education and research program that balances fundamental and applied aspects, preparing engineers to meet future industry challenges.
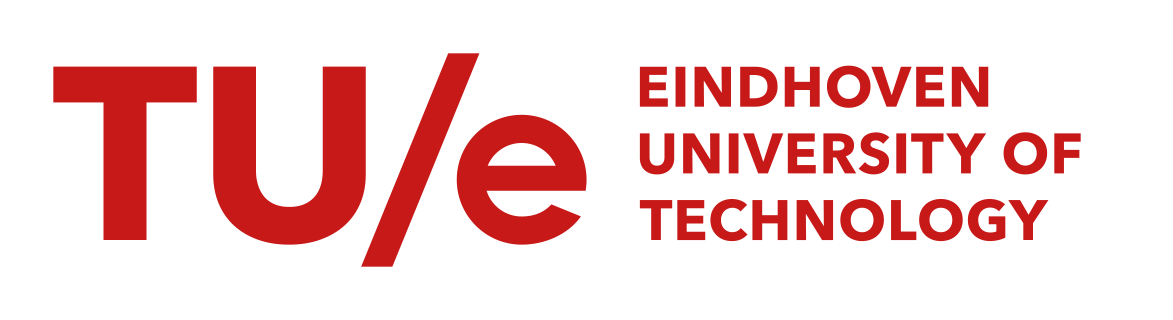
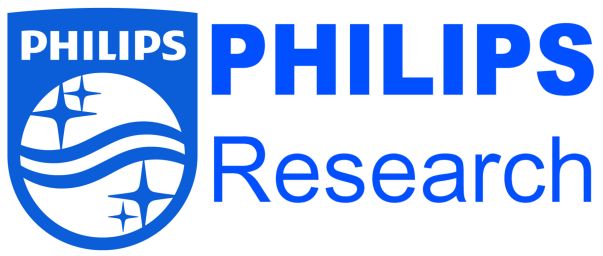
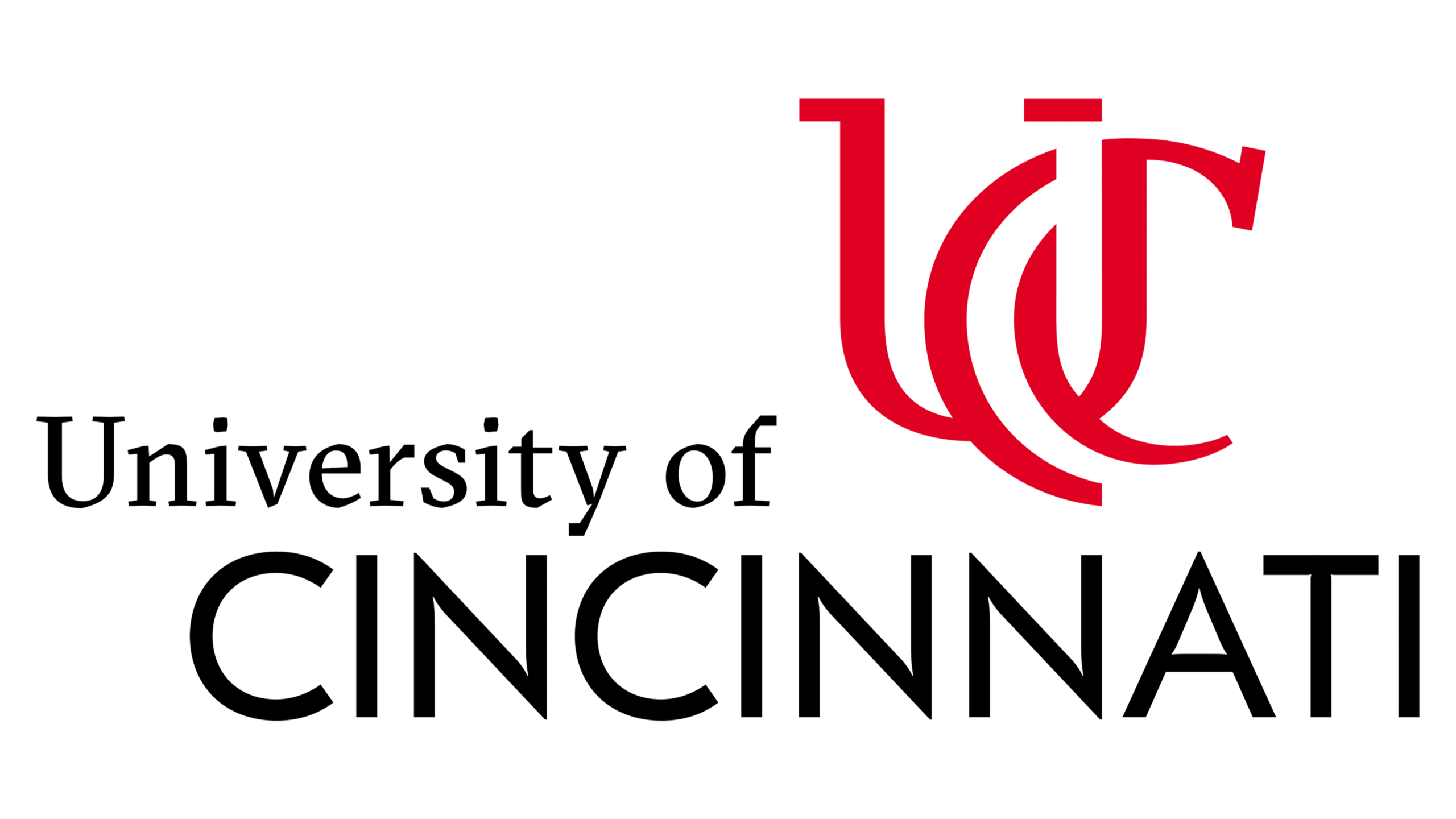
The Importance of Artificial Skin Platform Development
The Potential of Sweat
The main function of sweat is to thermoregulate the body, lowering its temperature when it rises. This sweat is secreted by sweat glands (the average person having 1.6 and 5 million) distributed over different areas. There are two types of glands (Figure 1) :
- Eccrine glands: located all over the body except for the lips, nails, and certain genital areas.
- Apocrine glands: located only where there is hair. Due to their location, they are composed of a mixture of sweat, sebum, proteins, lipids, etc. 1
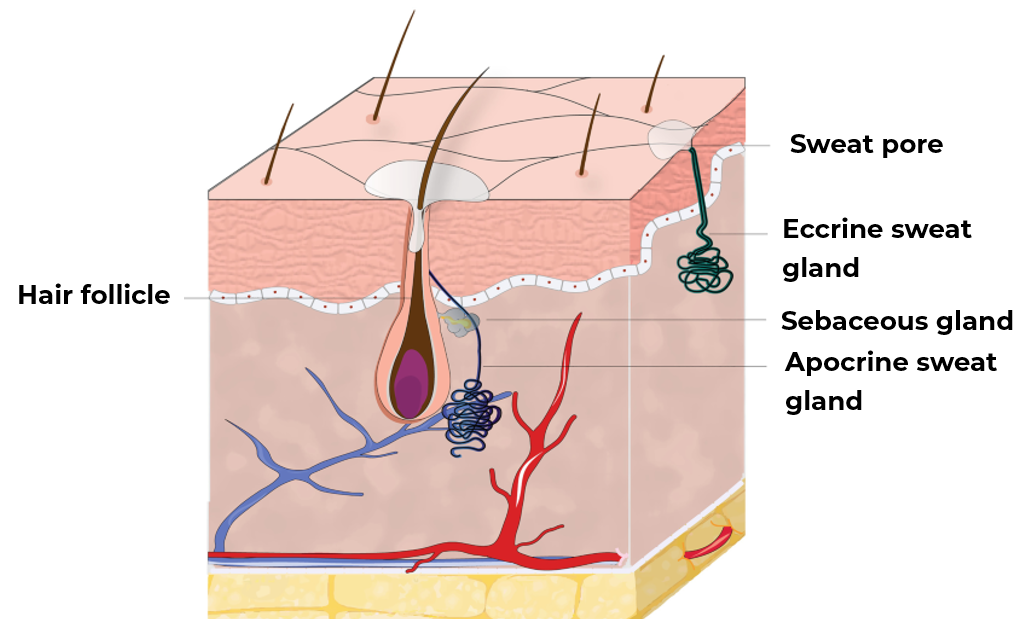
Figure 1: Sweat glands localization.2
Although the sweat excreted by these glands is 99% water, it remains rich in analytes, making it ideal for measuring biomarkers. In fact, sweat analysis overcomes problems associated with traditional analysis methods, such as blood analysis which requires invasive sampling. Interest in developing portable devices for measuring sweat biomarkers has increased since the 1950s in fields like infectious diseases, immunology, neurology, psychiatry, endocrinology, and more.3
What Are the Limits of Skin-Based Studies?
In order to develop portable systems or wearable sweat patches for measuring biomarker concentration, or even to test cosmetics such as deodorants or creams, in vivo or ex vivo skin-based studies are required.The skin is divided into three layers: the epidermis, dermis, and hypodermis. Each layer is made up of cell types and structures specific to their roles (Table 1).
Table 1: Characteristics of the three skin layers.4
Layer | Structure | Cell types | Extra cellular matrix components |
---|---|---|---|
Hypodermis | Blood vessels, nerves, hair follicles | Adipocytes, fibroblasts, endothelial cells, muscle cells | Elastine, type I collagen |
Dermis | Blood vessels, nerves, mechanoreceptors, appendages | Fibroblasts, endothelial cells, Langherans cells, muscle cells | Elastin, proteogylcans, type I, IV and VII collagens |
Epidermis | Stratifie keratinized epihelium | Keratinocytes, melanocytes, merkel cells, Langherans cells | Keratin, type IV and VII collagens |
Currently, most tests are performed on human or animal skin, but these methods have several drawbacks. For instance, in vivo or ex vivo tests can present various difficulties in terms of potential risks for the donor and may be ethically challenging. In addition, another major problem preventing the reliability and reproducibility of the results is the intra- and inter-human variability: skin and sweat differ according to zones, the donor age and activity (see Figure 2)4. For all these reasons, it was essential to develop platforms capable of providing reproducibility while mimicking skin characteristics with precision.
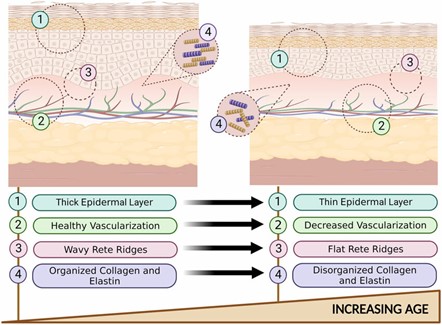
Figure 2: Histological changes in young skin VS old skin.5
Models to Mimic Human Skin
There are several types of models that mimic human skin for the above purposes. These include:
- Cell culture: air-liquid interface culture of keratinocytes on a dermal substitute to reconstitute the stratified layers of the skin.6 Culture can also result in the development of a single layer, known as 2D culture, which differs from the first stratified layer method, known as 3D culture (Table 2).
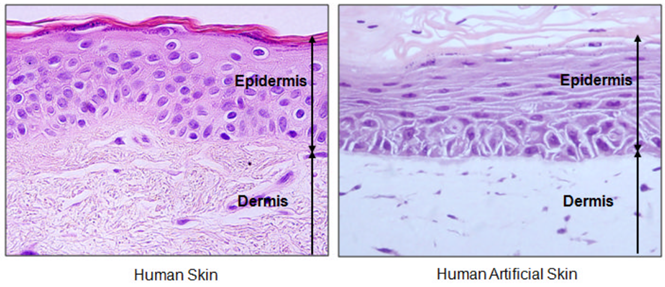
Figure 3: Optical microscopy of human or artificial skin.6
Table 2: Comparison of 2D and 3D cultures for skin models.4
2D Culture | 3D Culture |
---|---|
Single or co-cultured monolayers | Multilayered cultures |
reliminary studies on drug-cell interaction | Studies on complex drug-tissue interactions |
No air-liquid interface | Air-liquid interface |
Short cultivation times (days) | Long cultivation times (weeks) |
Low cost | High cost |
2. 3D bioprinting: use of biomaterials to reproduce a model equivalent to its counterpart for various applications (Figure 4).5
3. Microfluidics: This allows researchers to mimick skin with real cells for drug testing, known as skin-on-a-chip studies (Figure 5). Using microfluidics, users can imitate the topology of skin with sweat mechanisms. This technology can be modulated to mimic both mature and young skin, as well as all skin topologies, for optimal product development testing.
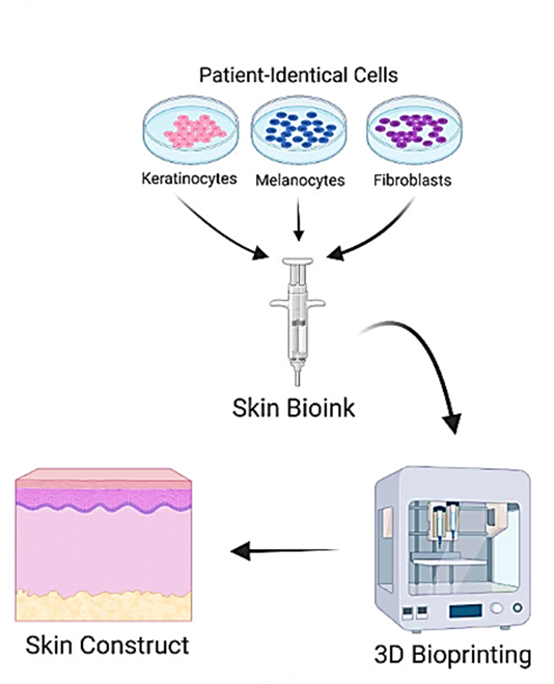
Figure 4: Process of 3D bioprinting human equivalent skin models (from 5).
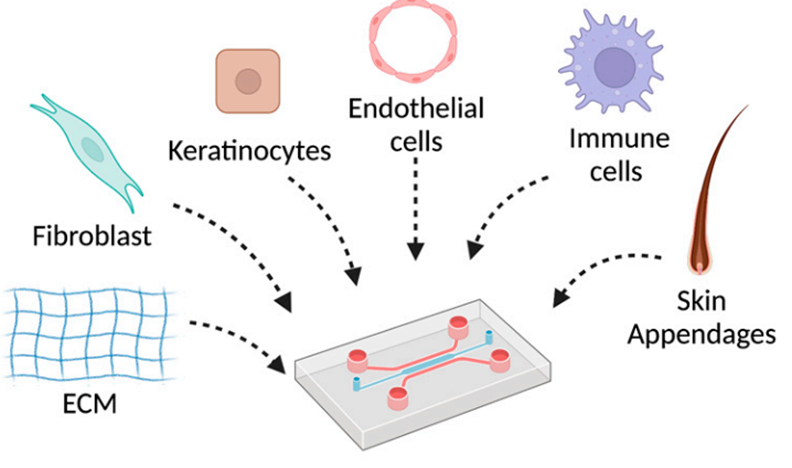
Figure 5: Skin-on-chip by addition of skin cells.7
Goal of the Study
To develop wearable sweat sensing patches, it’s critical to test them on skin with and without sweat. Previous studies have shown large inter-human variability in sweat and skin type. Hence, researchers at the Department of Mechanical Engineering planned on creating an artificial skin platform to avoid this variability by developing a stable, realistic, and adaptable platform.
In this paper, a microfluidic design was created to mimic skin perspiration using Fluigent’s flow controller and flow meter. The device precisely controlled the generation of perspiration into the platform according to the skin and human type.
Mimicking of Human Skin in a 3-Layer Platform
To improve medical and cosmetic research, a platform was designed to mimic human skin. This artificial skin platform is composed of three layers:
- 1. Reservoir layer: composed of several hexagonal chambers grouped together with channels to form different sections. Each section can be fed differently. The transparent resin layer was printed via Form SLA (Formlabs).
- 2. Membrane layer: features high hydrodynamic resistance to create a constant flow. The membrane was made of hydrophilic polycarbonate, and contained pores.
- 3. Skin layer: a layer of silicone rubber (Shore 15 – Resion Resin Technologies) molded onto the actual skin surface to be imitated.
The Fluigent MFCS series controller pressurized the liquid-containing tube which is connected to the reservoir layer sections via the Flow Unit XS flowmeter to precisely control liquid delivery within the platform. This precise control avoids pore obstruction and imitates sweat.
Artificial sweat pores were created using laser-guided molybdenum wires. They have a diameter of 60 µm, which is equivalent to natural pores from 50 to 80 µm.
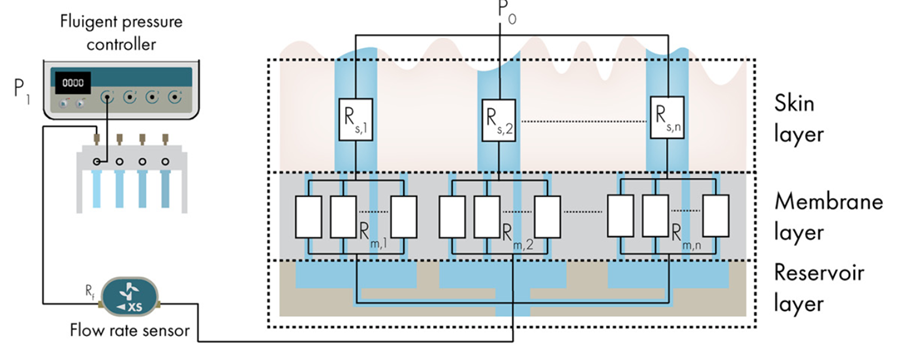
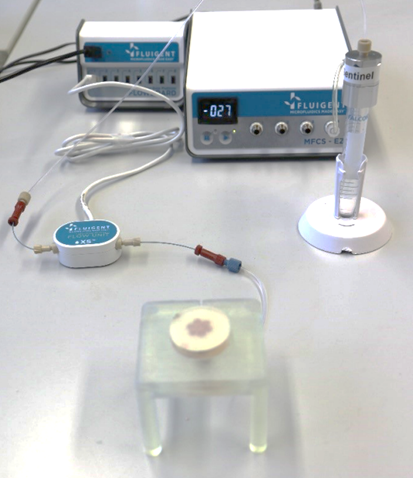
Figure 6: Schematic overview of the working principle of the artificial skin platform. P1 = pressure applied by the pressure controller, P0 = hydrostatic pressure due to the height difference between the top of the skin layer and the meniscus in the storage tube, Rf = hydrodynamic resistance of flow sensor, Rm = hydrodynamic resistance of open pores in membrane and Rs = hydrodynamic resistance of artificial sweat pores.
Several parameters of human sweating were tested such as wetting properties, pulsed sweat excretion with active and inactive periods, and large ranges of sweat delivery to verify the platform capacities.
The artificial skin platform was adapted according to the sweating location, particularly in terms of skin texture, wetting properties and sweat pore density (Figure 7).
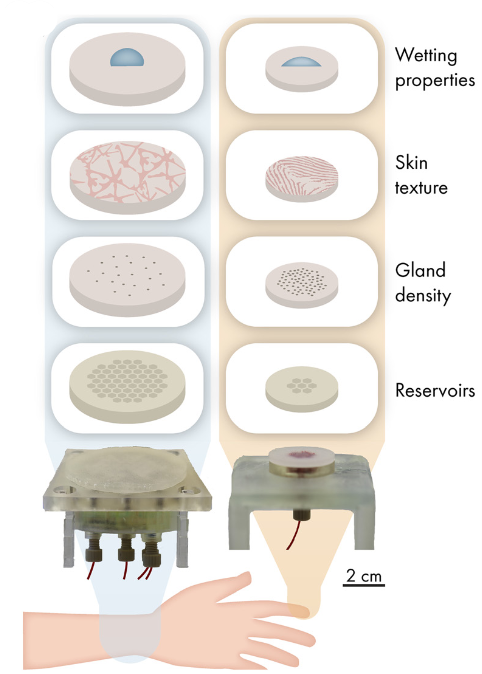
Figure 7: Overview of the adaptable components of the artificial skin platform to mimic different targeted body locations (left = arm and right = finger).
Partial Results: Artificial Skin Platform Permits Mimicking Human Perspiration
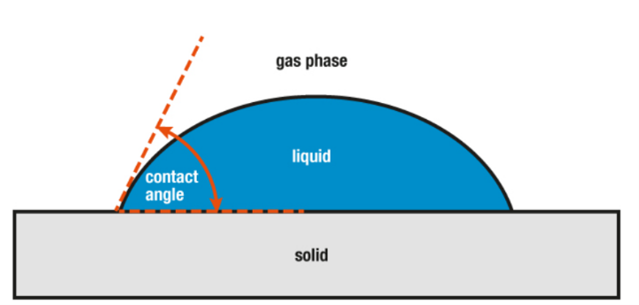
Figure 8: Contact angle explanation.8
The wetting properties of human and artificial skin were tested, and results were compared. This wetting property was studied by the contact angle measurements. The contact angle is defined as the angle formed between the skin and the liquid droplet (Figure 8).8
For human skin, the contact angle was measured on the skin of the arm or finger with either sweat or water. The results showed that there was a difference in the contact angle between different locations, with the contact angle being higher for the arm than for the finger. In addition, water or sweat secretion influences the contact angle, with a decrease of about 25° observed in the case of sweat versus water. These differences highlight the importance of considering wetting properties (Figure 9).
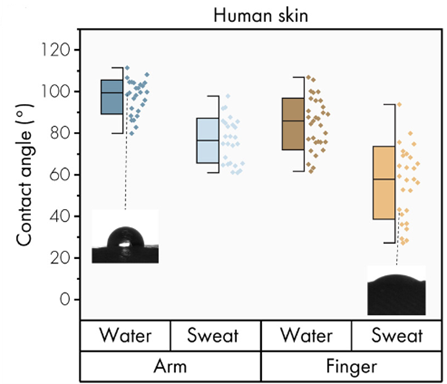
Figure 9: Wetting properties of human skin by contact angle measurements.
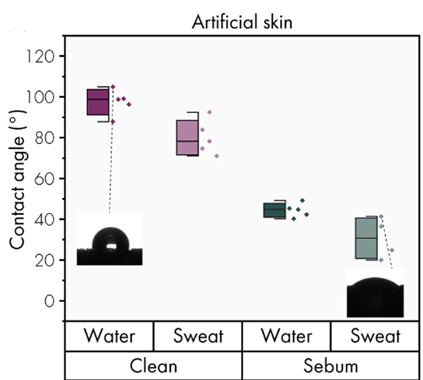
Figure 10: Wetting properties of artificial skin by contact angle measurements.
For artificial skin, the contact angle was measured for 1) clean skin and 2) skin with sebum. Natural skin is hydrophobic, but sebum secretion modifies the skin into hydrophilic, thus affecting the contact angle. In fact, “clean” skin is a characteristic of the one on the arm, whereas “sebum” skin presents characteristics close to the skin on the fingers. The results are similar to natural human skin, with a decrease in contact angle observed in the presence of sebum (Figure 10).
The study of droplet transpiration has shown that in sebaceous skin, droplets spread along the skin, whereas in clean skin, droplets don’t move. The behavior of sebum droplets is again like human skin (Figure 11).
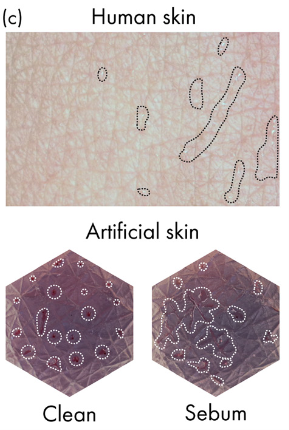
Figure 11: Visual qualification of dynamic wetting properties.
The second part of the study involves checking the platform’s ability to secrete sweat correctly, based on the flowmeter and pressure controller’s ability to accurately control it.
The results of the analytical model, which was based on an equation between pressure and hydrodynamic resistance, have been correlated with experimental results to predict expected flow at set pressure (Figure 12.A).
The flow rate was increased in 50 nL/min steps from 0 to 950 nL/min, followed by decreasing steps, to test the response efficiency and stability of the flowmeter for the artificial skin platform. The result showed that the platform generated the required flow rates with a stabilization time of less than 10 seconds, thanks to the precision of MFCS and the Flow Unit (Figure 12.B).
The platform was then tested for its ability to mimic human glands. Recent studies explained that human sweat glands in vivo excrete sweat in a pulsed way, with active and inactive periods lasting a few minutes9. The results showed that it is possible to reproduce these alternating periods of sweating and non-sweating with the platform, pausing for one minute and stabilizing in less than 10 seconds (Figure 12.C).
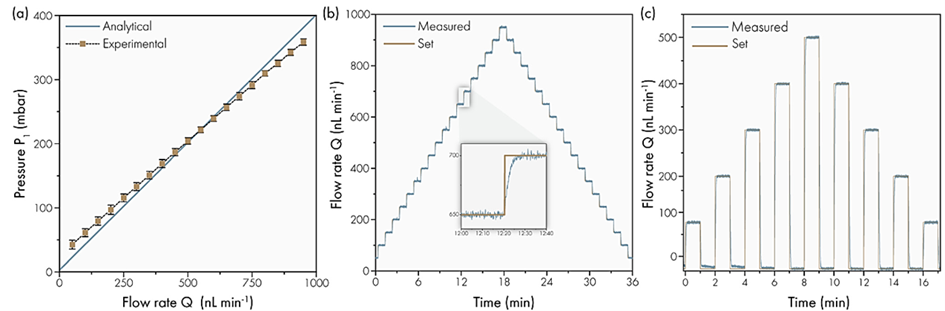
Figure 12: Overall dynamic flow behavior of artificial skin platform with 120 active sweat pores.
Finally, the artificial skin platform was evaluated in terms of secreting the full range of human perspiration. This range extended from 0.1-1 nL/min/pore of sweat for individuals at rest to 1-20 nL/min/pore for people in intense activity. The results showed that the artificial skin platform could cover the entire transpiration range, from 100 nL/min (0.8 nL/min/pore) to 1000 nL/min (8nL/min/pore) (Figure 13 and videos).
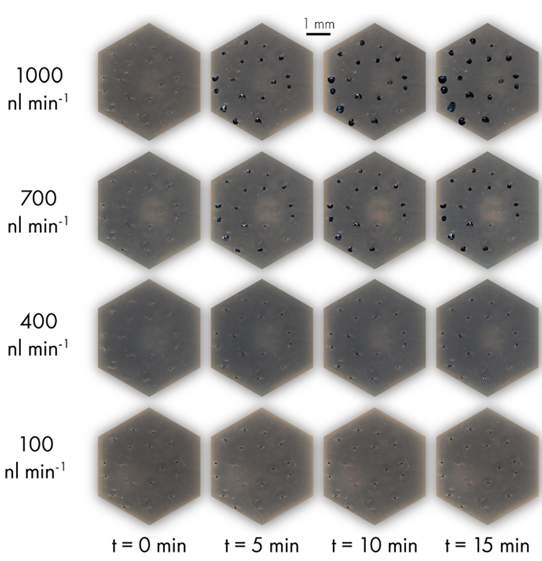
Figure 13: Vizualisation of droplet formation and flow through artificial skin.
Videos : Sweat secretion through the pores of the artificial platform at different sweat levels (left = 100 nL/min/pore, right = 500 nL/min/pore).
Conclusion
In this paper, researchers from the Philips Research, the Eindhoven University of Technology, and the University of Cincinnati presented a microfluidic platform to mimic skin comportment that could facilitate the development of wearable sweat sensing patches for biomarker diagnostics. The platform successfully mimicked the human perspiration variabilities and integrates Fluigent’s pressure pump, MFCS series, and flow meter Flow Unit XS to precisely imitate the sequential excretion of sweat and its level of perspiration. All results demonstrated that the artificial skin platform has wetting properties, skin topography and perspiration types that make it a potential platform for future innovation in this field.
Related products
Expertises & Resources
-
Microfluidics Case Studies Real-Time Monitoring Platform for Ocular Drug Delivery, Integrating Fluigent’s Flow EZ Read more
-
Microfluidics Case Studies Mimicking tumor microenvironment using a 3D microfluidic model to improve cancer investigations Read more
-
Expert Reviews: Basics of Microfluidics Pressure-Controlled Microfluidics in Organ-On-A-Chip Research Read more
References
1. Moonen, E. J. M. et al. Wearable sweat sensing for prolonged, semicontinuous, and nonobtrusive health monitoring. VIEW 1, 20200077 (2020).
2. Berth-Jones, J. & Tebbs, V. M. Disorders of Sweat Glands. in Atlas of Dermatology, Dermatopathology and Venereology (eds. Smoller, B. & Bagherani, N.) 1–18 (Springer International Publishing, Cham, 2020). doi:10.1007/978-3-319-45134-3_41-1.
3. Brasier, N. & Eckstein, J. Sweat as a Source of Next-Generation Digital Biomarkers. Digit. Biomark. 3, 155–165 (2019).
4. Moniz, T., Costa Lima, S. A. & Reis, S. Human skin models: From healthy to disease‐mimetic systems; characteristics and applications. Br. J. Pharmacol. 177, 4314–4329 (2020).
5. Ansaf, R. et al. 3D bioprinting—a model for skin aging. Regen. Biomater. 10, (2023).
6. Brohem, C. A. et al. Artificial skin in perspective: concepts and applications. Pigment Cell Melanoma Res. 24, 35–50 (2011).
7. Fernandez-Carro, E. et al. Modeling an Optimal 3D Skin-on-Chip within Microfluidic Devices for Pharmacological Studies. Pharmaceutics 14, 1417 (2022).
8. Angle de contact: Définition, Sens et de l’équipement de mesure. https://www.linseis.com/fr/grandeurs-mesurees/angle-de-contact/.
9. Chen, X., Gasecka, P., Formanek, F., Galey, J.-B. & Rigneault, H. In vivo single human sweat gland activity monitoring using coherent anti-Stokes Raman scattering and two-photon excited auto-fluorescence microscopy. Br. J. Dermatol. 174, (2015).