The Importance of Flow Control Stability in Microfluidics
Stable flow rates are vital for accurate and reproducible results in microfluidics. This review compares different flow control systems (syringe pumps, peristaltic pumps, and pressure-based controllers) focusing on their performance in terms of stability and response times. It highlights the importance of stable flow in various microfluidic applications, including imaging, high-precision manipulation, cell sorting, and lipid nanoparticle production. In each case, optimized flow control significantly enhances experimental reliability, precision, and reproducibility.
Why Flow Control Stability Matters in Microfluidics?
Though microfluidics was initially developed for applications in analytical chemistry and physics, it has since expanded into biology, medicine, and materials science.1,2 Today, microfluidic technologies are not only advancing research but are also part of everyday life, powering devices like inkjet printers and diagnostic tests.3–6
While the microfluidic chip often receives the most focus, the performance of the overall system depends heavily on the control of fluid flow (Figure 1). In many systems, stable and precise flow control is essential. Variations in flow can impact experimental outcomes, reducing the reliability and reproducibility of results.7
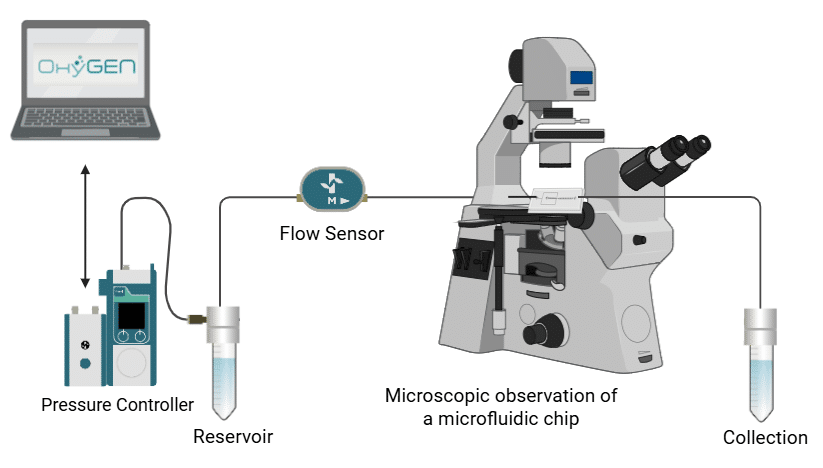
This review explores the importance of flow control stability in microfluidics. We define key parameters such as response time and resolution, assess common strategies for flow generation and regulation, and finally illustrate the role of flow stability with applications from different fields.
Key Parameters for Assessing Flow Control and Stability
Flow control stability in microfluidics refers to the ability of a system to maintain a prescribed flow rate or pressure with minimal deviation over time. Due to the sensitivity of microscale systems to even minor perturbations, precise control is essential to ensure experimental reproducibility and reliability.
Flow stability is commonly assessed using classical statistical indicators such as range, standard deviation (SD), and coefficient of variation (CV), all of which are directly applicable in microfluidic contexts. The range captures the span between the maximum and minimum values. The standard deviation provides a more representative measure of variability across time, while the CV, the ratio of SD to the mean, offers a normalized, dimensionless value, enabling comparison across systems operating at different flow rates.
Beyond these steady-state metrics, dynamic parameters play a critical role in evaluating how quickly and accurately a flow-handling instrument can respond to changes.
- Response time refers to the time between the sending of a command (keystroke) and the initial reaction of the system, such as the onset of fluid movement (Figure 2a).
- Rising time denotes the duration required for the system to increase from 10% to 90% of the target value, reflecting the speed of actuation (Figure 2b).
- Settling time is the total time needed for the system to reach and remain within a predefined error margin (typically ±5%) around the target value, encompassing both initial response and final stabilization (Figure 2c).
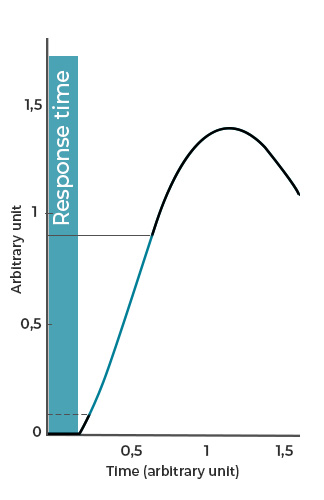
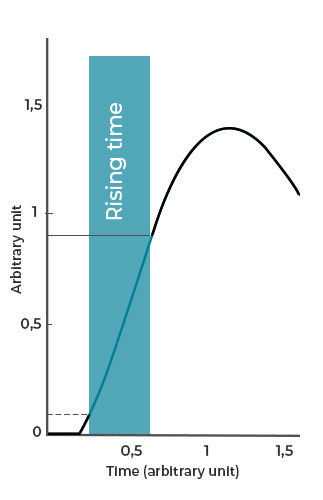
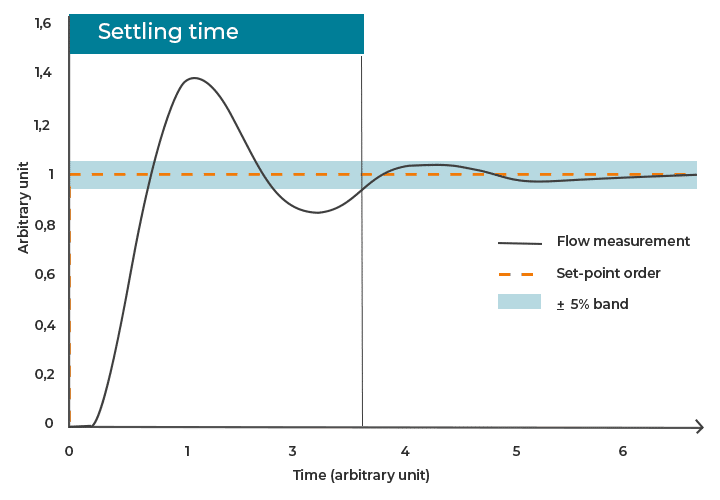
Figure 2: Examples of a) response time, b) rising time, and c) settling time of flow-handling instrument.
The shorter the response time, the faster the flow-handling instrument can execute its feedback loop to compensate for any variations in the quantity of interest; thus, enhancing flow stability.
- Resolution refers to the smallest detectable change in the physical quantity (flow rate in our case) that a sensor can measure, or the smallest increment a controller can apply.
Higher resolution allows the system to more accurately track input commands and finely regulate output, contributing significantly to flow stability.
Together, these parameters provide a comprehensive framework for characterizing both the static and dynamic aspects of flow control stability in microfluidic systems. This is essential for ensuring precision in increasingly complex and time-sensitive applications.
Comparative Overview of Flow Control Instruments in Microfluidics
A variety of flow control systems are used in microfluidics, each with different mechanisms and performance characteristics. Among the most widely employed are syringe pumps, peristaltic pumps, and pressure-based flow controllers. While each has specific advantages, their ability to maintain stable, accurate, and responsive flow is a key factor for ensuring precision in microfluidic applications.
- With Syringe pumps a motor-driven plunger pushes fluid from a syringe into the microfluidic system. They have been used due to their ease of operation and accessibility. Their stepper motor actuation introduces pulsatile flow and results in relatively long response times, especially when adjusting flow rates or executing dynamic protocols in systems with resistance to flow. These characteristics can limit flow stability, particularly in experiments requiring fine control or rapid transitions.
- Peristaltic pumps compress flexible tubing segments with rotating rollers, generating a forward-moving wave that drives fluid flow. This mechanism enables continuous, non-contact fluid transport and minimizes the risk of cross-contamination, making them well-suited for handling sensitive or hazardous fluids. However, intrinsic to their actuation principle is pulsatile flow, characterized by periodic fluctuations in flow rate. These pulsations can become particularly pronounced at low flow regimes or in microfluidic systems with high sensitivity to transient shear forces and pressure variations, potentially compromising experimental precision and reproducibility.
- Pressure-based flow controllers deliver fluid by regulating the pressure applied to a fluid reservoir. This provides pulse-free, highly responsive, and stable flow control. These systems can be coupled with feedback algorithms that enable users to set a target flow rate, with the applied pressure continuously and dynamically adjusted to maintain the desired flow.This compensates for variations in system resistance or fluid properties. An accurate closed-loop flow control system often requires the integration of a flow rate sensor. This approach offers fast response times and stability, making pressure controllers especially advantageous for high-precision and time-sensitive microfluidic workflows.
In the following graphs (Figures 3-4), we present a comparison between Fluigent pressure-based controllers (Flow-EZTM), syringe pumps, and peristaltic pumps in terms of flow stability.
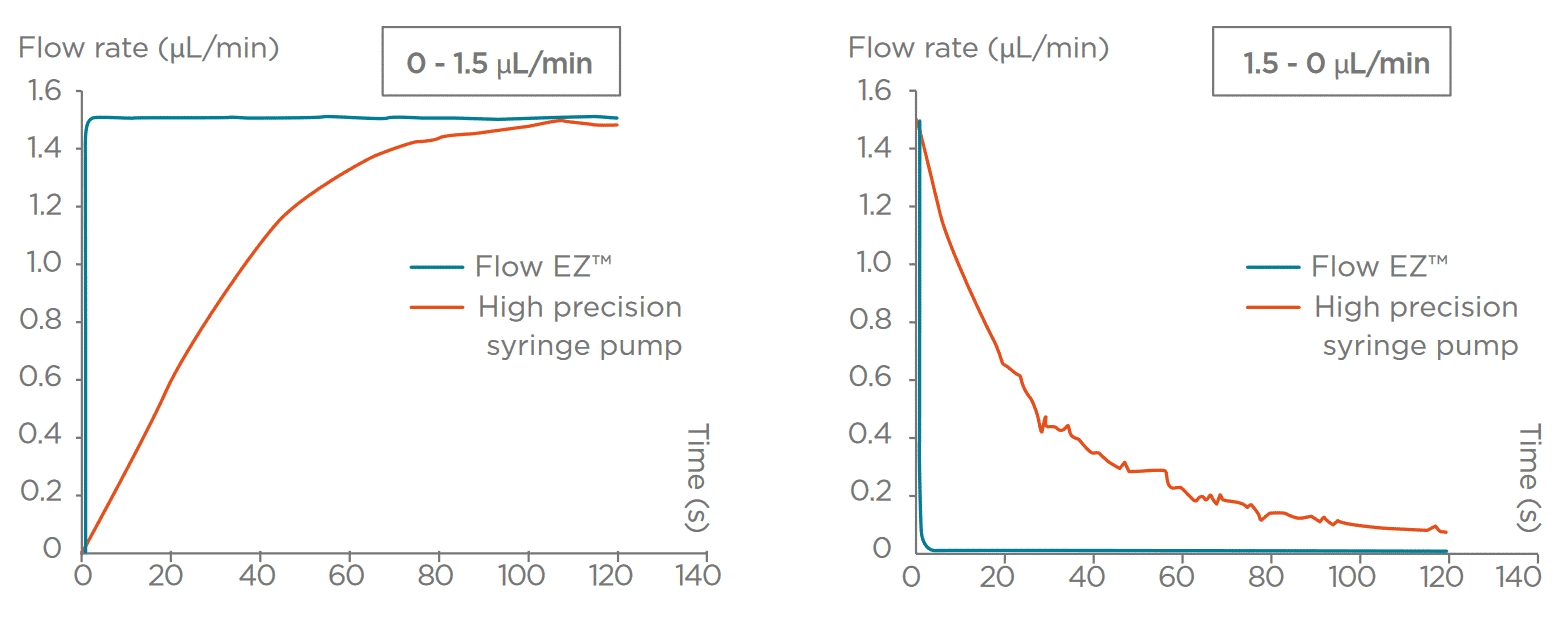
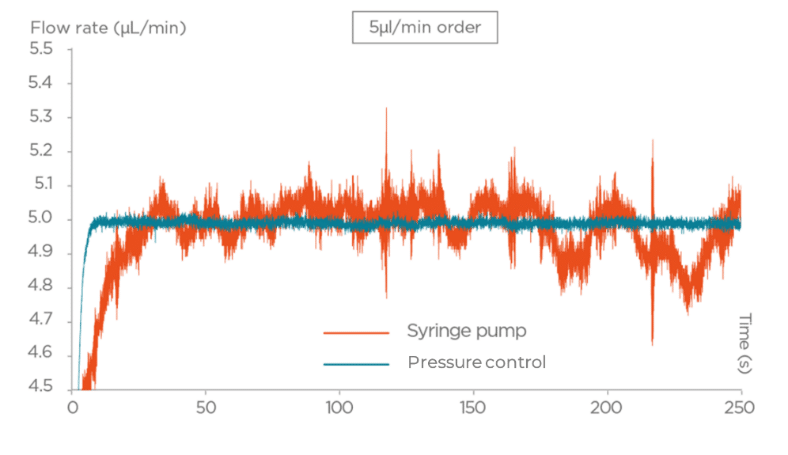
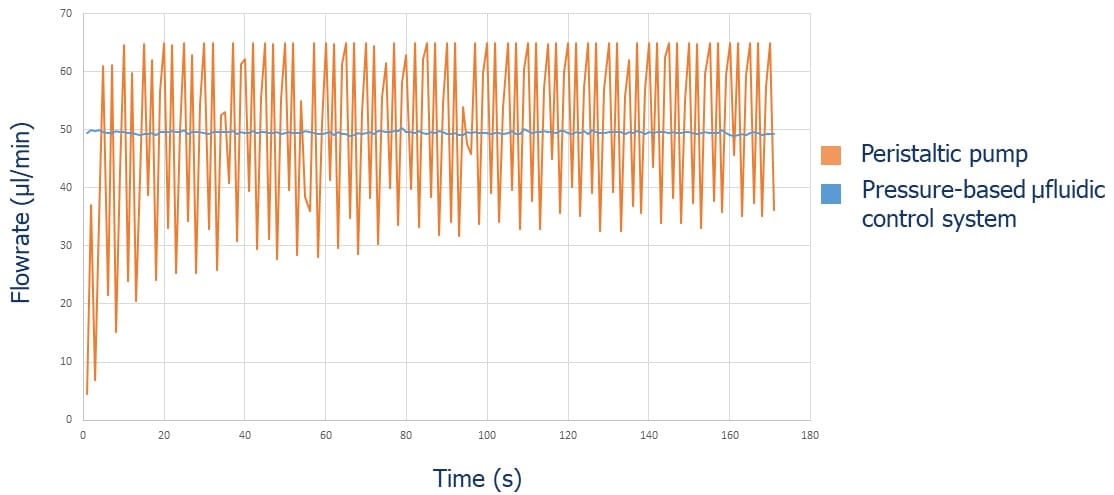
Examples of Applications Influenced by Flow Stability
At a micrometric scale, flow control stability is critical across a wide range of applications, directly influencing the reproducibility, and interpretability of experimental outcomes. Whether for imaging, biological manipulation, or particle processing, stable flow ensures that microfluidic conditions remain consistent, minimizing variability and enhancing data reliability. The following examples illustrate how precise flow regulation provides optimal performance across several key microfluidic techniques.
1. Imaging techniques
In imaging applications, flow stability is essential for producing accurate and interpretable visual data. Laser Speckle Contrast Imaging (LSCI), for instance, is used to assess blood flow dynamics. Syringe pump-induced flow fluctuations can be misinterpreted as biological variation, reducing result fidelity. Pressure-based flow controllers ensure consistent flow rates. In this case study, pressure-driven systems enhanced flow reproducibility and eliminated artifacts in LSCI, significantly improving the reliability of high-resolution imaging (Figure 5-6).8
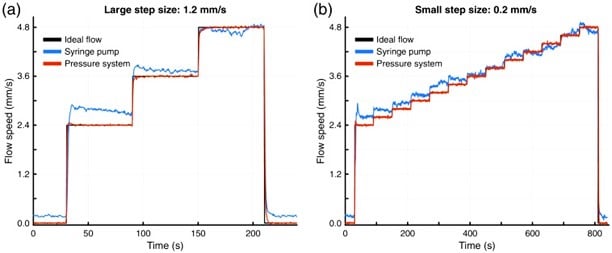
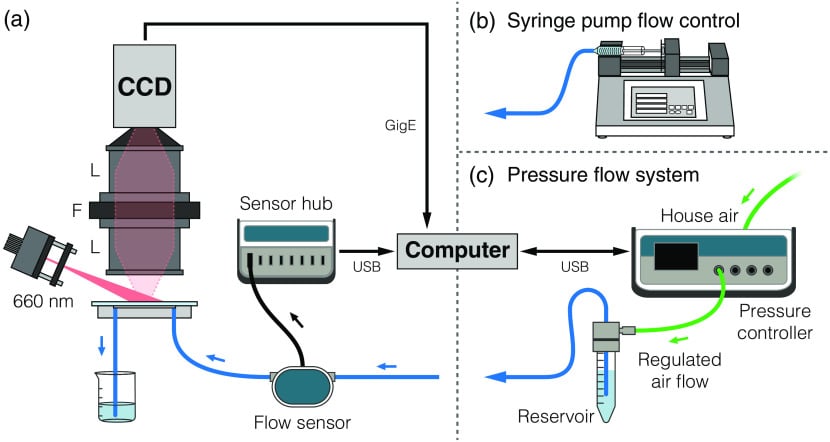
Figure 5: a) Flowrate control between syringe and pressure system b) Microfluidic setup for LSCI Microscopy (Sullender, C. T. et al. J. Biomed. Opt. 2023, 28 (03)).
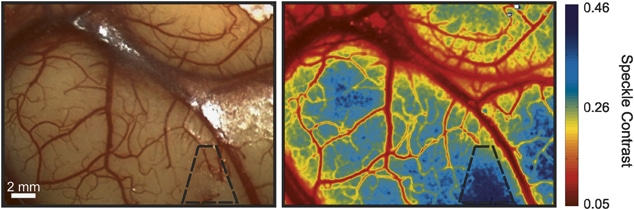
Figure 6: Example of a human cortex captured by a surgical microscope and the corresponding speckle contrast image (Sullender, C. T. et al. J. Biomed. Opt. 2023, 28 (03)).
2. Microscopy
In Liquid Phase Transmission Electron Microscopy (LPTEM), precise control of fluid flow is critical for high-resolution imaging of samples in liquid environments. Integrating microfluidics with LPTEM regulates the flow of reagents through the imaging area, ensuring that the liquid is consistently directed between the chips and into the observation zone (Figure 7). This setup maintains uniform temperature and environmental conditions and allows for the real-time study of dynamic processes, such as copper electrodeposition, without compromising the integrity of the sample (Figure 8). The control provided by pressure-driven systems is essential for obtaining clear, reproducible imaging at the nanoscale, offering valuable insights into phenomena that depend on consistent fluid movement for accurate analysis.
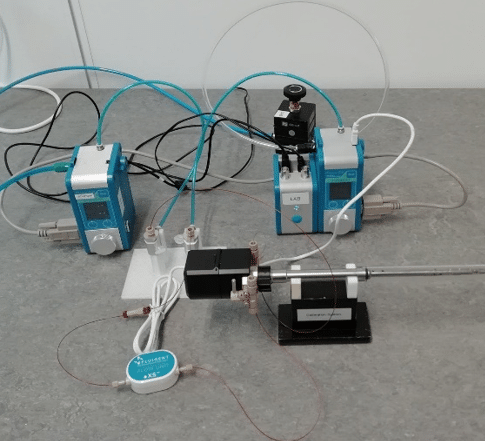

Figure 8: Time series of images showing the growth and etching process of Cu dendrites on the Pt electrode using microfluidics.
3. High-Precision Micro-cannulas Manipulation
In developmental biology and physiological studies, such as zebrafish heart analysis, flow control stability ensures accurate modulation of mechanical loads. In a case study (Dalhousie University), micro-cannulas were inserted into zebrafish embryos to inject saline upstream of the heart, inducing atrial dilation. Using pressure-based flow control allowed researchers to observe changes in heart rate and stroke area in real time (Figures 9-10). This approach revealed critical insights into mechano-mechanical coupling during early cardiac development and demonstrated how precise, stable flow is essential for in vivo manipulation at micro scales.9
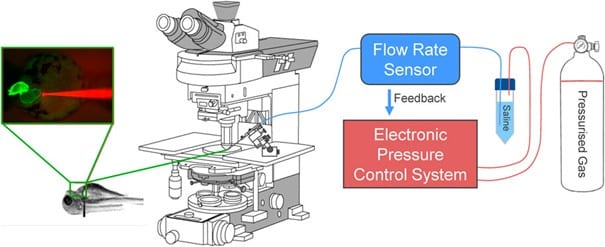
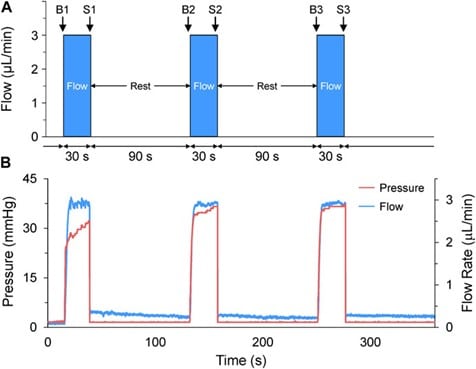
In C. elegans experiments, pressure-controlled systems facilitate gentle and consistent immobilization or reagent delivery, minimizing stress and mechanical disturbance to the organism. This improves the reliability of imaging, behavioral tracking, and neuronal stimulation protocols. 10,11
4. Cell Sorting
For cell sorting and particle separation, flow control stability determines the accuracy and resolution of sorting outcomes. Microfluidic single-cell sorters rely on consistent flow to direct cells based on size or markers. In an experiment using a spiral-shaped microfluidic device, pressure-based controllers enabled separation of 7.3 µm and 15 µm particles by maintaining defined flow conditions (Figures 11-12). The resulting high sorting fidelity was achieved through fine control of inertial forces.
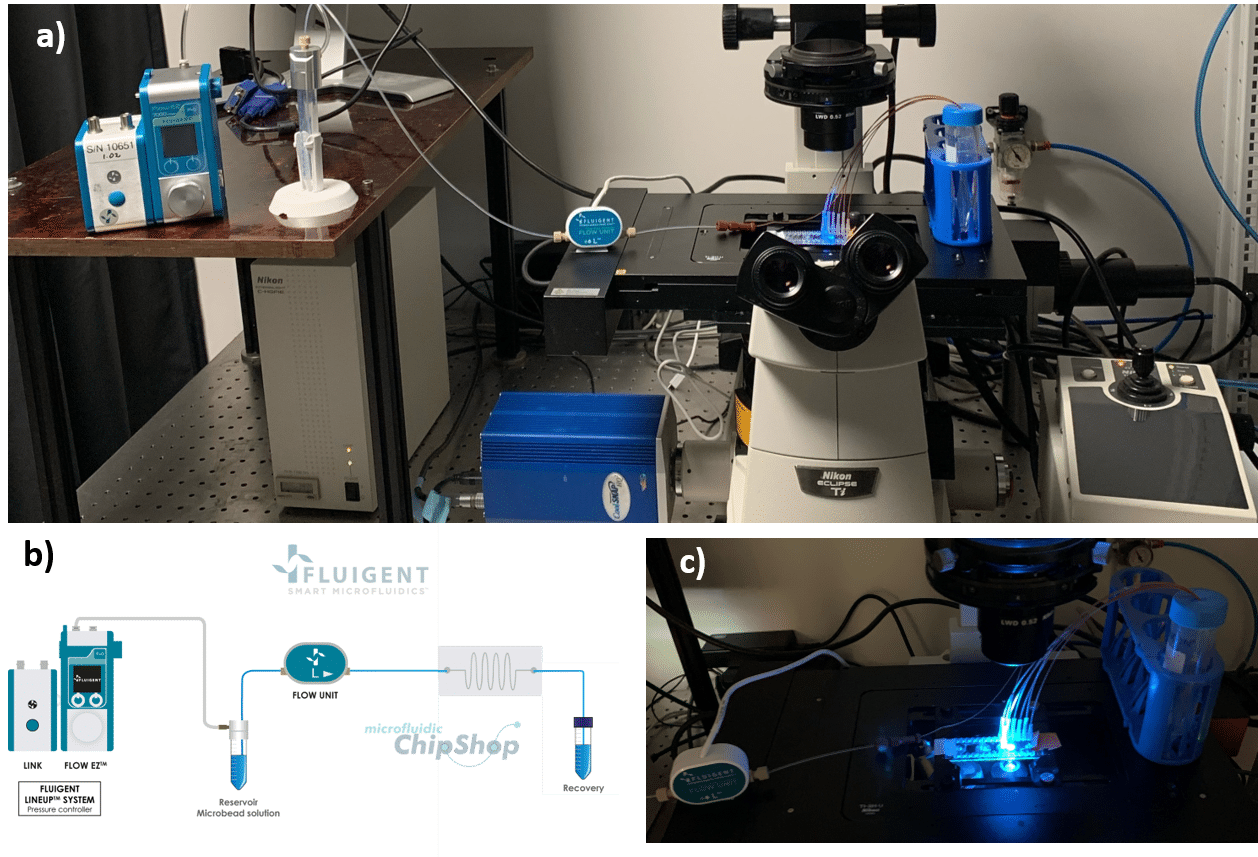
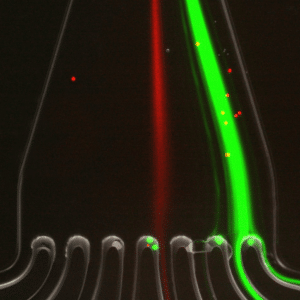
5. Lipid Nanoparticle (LNP) Production
In the development of drug delivery, flow control stability plays a crucial role in determining particle size and uniformity.12–14 In this case study (from the Ca’Foscari University of Venice), researchers used microfluidic mixers to produce amiodarone-loaded liposomes by adjusting the flow rate ratio (FRR) between aqueous and organic phases. Pressure-based flow controllers maintained a constant total flow rate (TFR) and precisely modulated the FRR, yielding liposomes with desirable size distributions and polydispersity indices (Figures 13-14). Stable flow was essential to ensuring consistency across batches, ultimately impacting therapeutic performance and reproducibility. 15
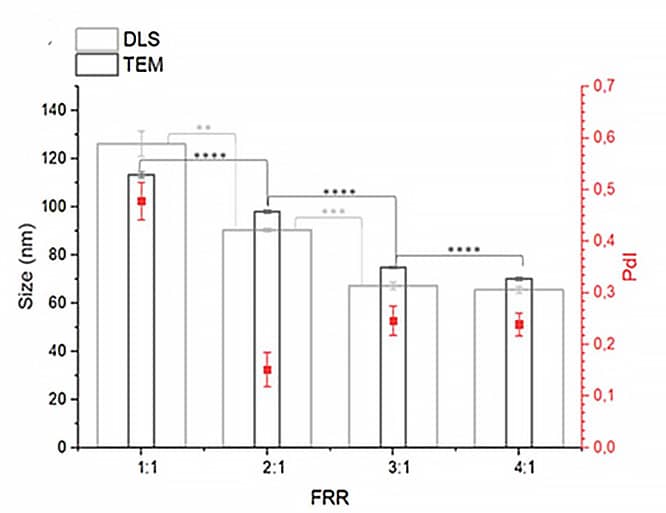
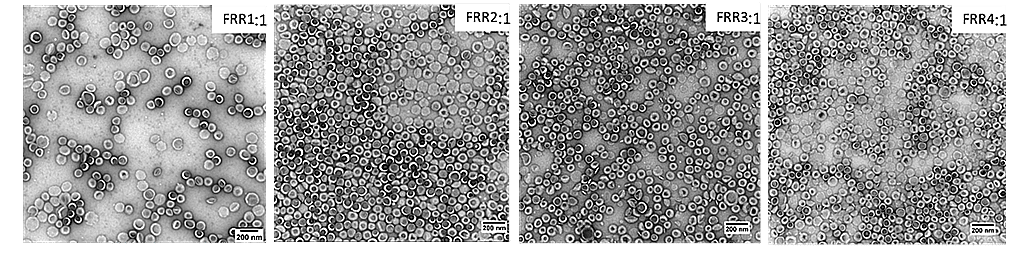
6. Organ-on-a-Chip (OOC)
OOC systems require controlled liquid flow to mimic physiological microenvironments and support long-term cell viability and function. This is particularly important in gut-on-a-chip platforms and other systems, where fluid-induced shear stress regulates epithelial differentiation, barrier integrity, and microbial interactions. As previously explained, traditional methods such as peristaltic pumps often introduce flow fluctuations at low rates and require frequent recalibration. Pressure-based flow controllers offer accurate, pulse-free flow delivery and rapid responsiveness. When applied to gut-on-chip models, control enables the formation of uniform epithelial monolayers under physiologically relevant conditions, making the system suitable for studying intestinal function. Controlled flow permits the generation of robust and reproducible tissue models, such as monolayers, that are directly usable for Organ-on-a-Chip applications (Figure 14).
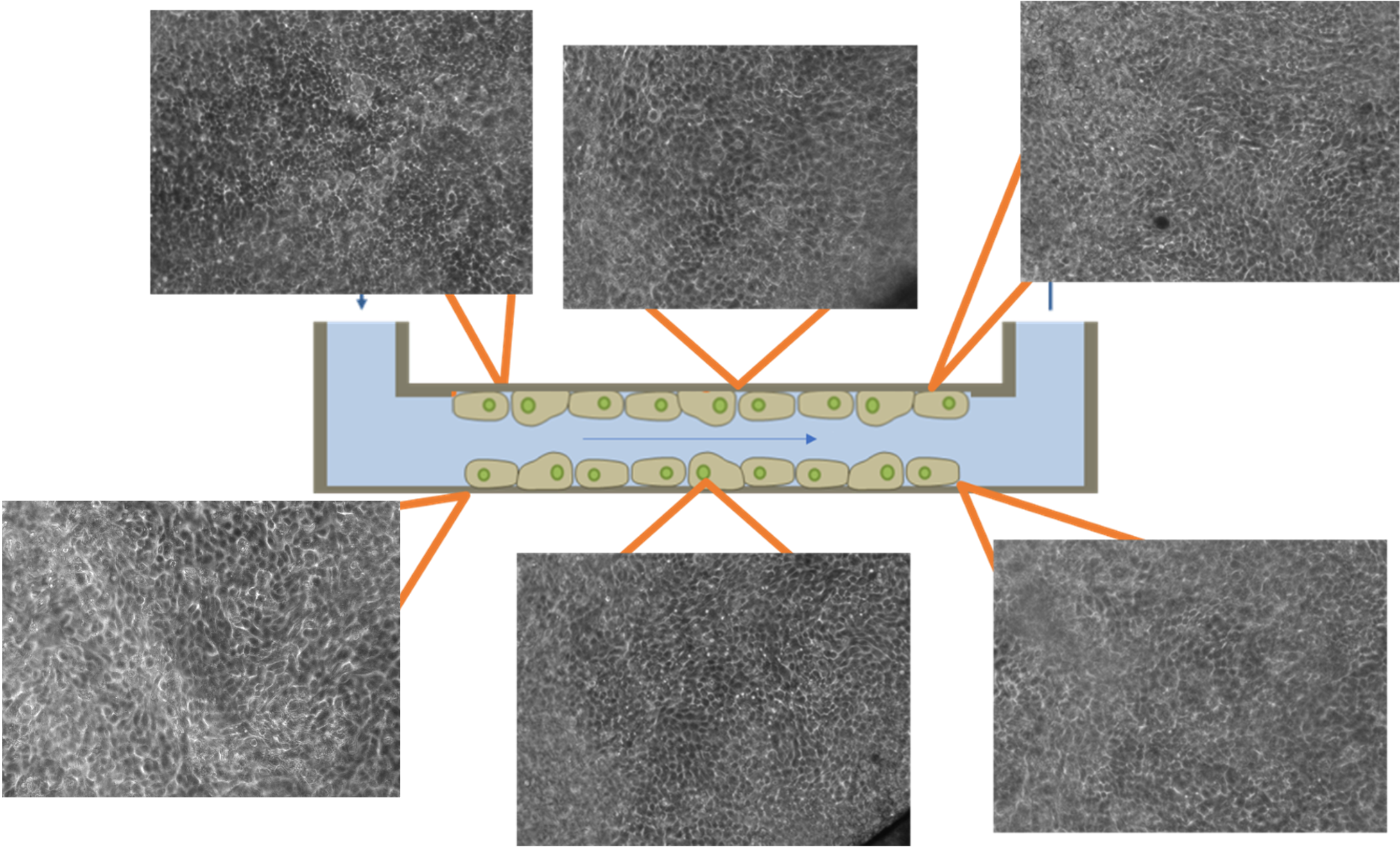
Conclusion
We have highlighted the critical role of flow control stability in microfluidic systems and its impact on many diverse applications including microscopy, high-precision manipulation, cell sorting, lipid nanoparticle production, and OOC. We compared pressure-based flow controllers to syringe pumps and peristaltic pumps, showing how pressure-driven systems provide superior stability and accuracy. Continued advancements in flow control technologies will further refine microfluidic applications, opening new possibilities for high-precision research and innovation across multiple disciplines.
👉 Ready to improve flow control stability in your setup? Contact our experts or explore our microfluidic pressure control systems.
Related content
Resources & Expertises
References
1. Whitesides, G. M. The origins and the future of microfluidics. Nature 442, 368–373 (2006).
2. Battat, S., Weitz, D. A. & Whitesides, G. M. An outlook on microfluidics: the promise and the challenge. Lab. Chip 22, 530–536 (2022).
3. Zub, K., Hoeppener, S. & Schubert, U. S. Inkjet Printing and 3D Printing Strategies for Biosensing, Analytical, and Diagnostic Applications. Adv. Mater. 34, 2105015 (2022).
4. Su, W., Cook, B. S., Fang, Y. & Tentzeris, M. M. Fully inkjet-printed microfluidics: a solution to low-cost rapid three-dimensional microfluidics fabrication with numerous electrical and sensing applications. Sci. Rep. 6, 35111 (2016).
5. Iyer, V., Yang, Z., Ko, J., Weissleder, R. & Issadore, D. Advancing microfluidic diagnostic chips into clinical use: a review of current challenges and opportunities. Lab. Chip 22, 3110–3121 (2022).
6. Wang, X. et al. Microfluidics-based strategies for molecular diagnostics of infectious diseases. Mil. Med. Res. 9, 11 (2022).
7. Cavaniol, C., Cesar, W., Descroix, S. & Viovy, J.-L. Flowmetering for microfluidics. Lab. Chip 22, 3603–3617 (2022).
8. Sullender, C. T. et al. Using pressure-driven flow systems to evaluate laser speckle contrast imaging. J. Biomed. Opt. 28, 036003 (2023).
9. Baillie, J. S., Gendernalik, A., Garrity, D. M., Bark, D. & Quinn, T. A. The in vivo study of cardiac mechano-electric and mechano-mechanical coupling during heart development in zebrafish. Front. Physiol. 14, (2023).
10. Bruggeman, C. W., Haasnoot, G. H. & Peterman, E. J. G. Microfluidics and fluorescence microscopy protocol to study the response of C. elegans to chemosensory stimuli. STAR Protoc. 4, 102121 (2023).
11. Rahimpouresfahani, F., Tabatabaei, N. & Rezai, P. High-throughput light sheet imaging of adult and larval C. elegans Parkinson’s disease model using a low-cost optofluidic device and a fluorescent microscope. RSC Adv. 14, 626–639 (2024).
12. Jaradat, E., Weaver, E., Meziane, A. & Lamprou, D. A. Microfluidics Technology for the Design and Formulation of Nanomedicines. Nanomaterials 11, 3440 (2021).
13. Jaradat, E., Weaver, E., Meziane, A. & Lamprou, D. A. Synthesis and Characterization of Paclitaxel-Loaded PEGylated Liposomes by the Microfluidics Method. Mol. Pharm. 20, 6184–6196 (2023).
14. Ghodke, J. et al. The Manufacturing and Characterisation of Eugenol-Enclosed Liposomes Produced by Microfluidic Method. Foods 12, 2940 (2023).
15. Saorin, A. et al. Microfluidic production of amiodarone loaded nanoparticles and application in drug repositioning in ovarian cancer. Sci. Rep. 14, 6280 (2024).